Help | Advanced Search

Condensed Matter > Materials Science
Title: element-specific ultrafast lattice dynamics in fept nanoparticles.
Abstract: Light-matter interaction at the nanoscale in magnetic alloys and heterostructures is a topic of intense research in view of potential applications in high-density magnetic recording. While the element-specific dynamics of electron spins is directly accessible to resonant x-ray pulses with femtosecond time structure, the possible element-specific atomic motion remains largely unexplored. We use ultrafast electron diffraction to probe the temporal evolution of lattice Bragg peaks of FePt nanoparticles embedded in a carbon matrix following excitation by an optical femtosecond laser pulse. The diffraction interference between Fe and Pt sublattices enables us to demonstrate that the Fe mean-square vibration amplitudes are significantly larger that those of Pt as expected from their different atomic mass. Both are found to increase as energy is transferred from the laser-excited electrons to the lattice. Contrary to this intuitive behavior, we observe a laser-induced lattice expansion that is larger for Pt than for Fe atoms during the first picosecond after laser excitation. This effect points to the strain-wave driven lattice expansion with the longitudinal acoustic Pt motion dominating that of Fe.
Submission history
Access paper:.
- Other Formats

References & Citations
- Google Scholar
- Semantic Scholar
BibTeX formatted citation

Bibliographic and Citation Tools
Code, data and media associated with this article, recommenders and search tools.
- Institution
arXivLabs: experimental projects with community collaborators
arXivLabs is a framework that allows collaborators to develop and share new arXiv features directly on our website.
Both individuals and organizations that work with arXivLabs have embraced and accepted our values of openness, community, excellence, and user data privacy. arXiv is committed to these values and only works with partners that adhere to them.
Have an idea for a project that will add value for arXiv's community? Learn more about arXivLabs .
Thank you for visiting nature.com. You are using a browser version with limited support for CSS. To obtain the best experience, we recommend you use a more up to date browser (or turn off compatibility mode in Internet Explorer). In the meantime, to ensure continued support, we are displaying the site without styles and JavaScript.
- View all journals
- My Account Login
- Explore content
- About the journal
- Publish with us
- Sign up for alerts
- Open access
- Published: 16 January 2020
Synthesis and Characterization of Selenium Nanoparticles-Lysozyme Nanohybrid System with Synergistic Antibacterial Properties
- Mahsa Vahdati 1 &
- Tahereh Tohidi Moghadam 2
Scientific Reports volume 10 , Article number: 510 ( 2020 ) Cite this article
26k Accesses
147 Citations
Metrics details
- Nanoparticles
- Nanoscale biophysics
This article has been updated
In the light of promising potency of selenium nanoparticles in biomedical applications, this is the first study to report the synergistic antibacterial activity of these nanoparticles and lysozyme. The nanohybrid system was prepared with various concentrations of each component. Resistance of Escherichia coli and Staphylococcus aureus was compared in the presence of individual Nano and Bio counterparts as well as the nanohybrid system. Upon interaction of SeNPs with Lysozyme, the nanohybrid system efficiently enhanced the antibacterial activity compared to the protein. Therefore, SeNPs play an important role in inhibition of bacterial growth at very low concentrations of protein; whereas very high amount of the protein is required to inhibit bacterial growth individually. On the other hand, lysozyme has also played a vital role in antibacterial property of SeNPs, inducing 100% inhibition at very low concentration of each component. Hence, presence of both nano and bio counterparts induced vital interplay in the Nanohybrid system. The aged samples also presented good stability of SeNPs both as the intact and complex form. Results of this effort highlight design of nanohybrid systems with synergistic antibacterial properties to overcome the emerging antibiotic resistance as well as to define fruitful applications in biomedicine and food safety.
Similar content being viewed by others
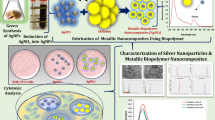
Biosynthesis of silver nanoparticles for the fabrication of non cytotoxic and antibacterial metallic polymer based nanocomposite system
Sadaf Raza, Asma Ansari, … Afsheen Aman
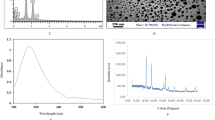
Niosomes-loaded selenium nanoparticles as a new approach for enhanced antibacterial, anti-biofilm, and anticancer activities
Abbas Haddadian, Farnoush Falahi Robattorki, … Amir Mirzaie
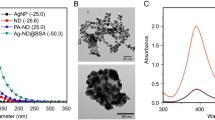
Nanodiamond-supported silver nanoparticles as potent and safe antibacterial agents
Be-Ming Chang, Lei Pan, … Huan-Cheng Chang
Introduction
There has been increasing concern in the era of antibiotic resistance as bacteria rapidly continue to develop adaptive countermeasures against conventional antibiotics 1 . Bacteria are potentially life-threatening agents, capable of promoting infectious diseases. The history of bacteria acting as causative agents for infection goes back to the 14 th century. Salvarsan was the first antimicrobial agent introduced in 1910. Soon after that, other antimicrobial agents such as chloramphenicol, nalidixic acid, and macrolides were used worldwide. The 20 th century experienced temporary relief to infectious bacterial pathogens. Nevertheless, overexposure to antibiotics and evolution of effective countermeasures against antibacterial agents led to the emergence of antibiotic-resistant bacteria 2 . Since then, significant efforts were focused on overcoming the emergence of these resistant strains by development of new antibiotic drugs boasting chemical diversity and identification of antibiotic-producing bacteria as well as additional antibiotics from natural, previously unexplored sources. Nonetheless, these advancements could not compensate the rapidly increasing number of resistant bacterial strains. Nanotechnology is widely used in the generation of diverse products in the fields of biology and medicine. Using nanotechnology in biology has provided many opportunities in many areas, including tissue engineering, drug delivery, diagnosis, imaging and fight against bacterial infections 3 . With the need for new antimicrobial agents, nanoparticles have been proposed to treat infections as they use different mechanisms to kill bacteria than conventional antibiotics 4 , with relatively low toxicity in human cells. As a result, Nanomaterials can be considered as a promising alternative to antibiotics to control bacterial infections 5 .
Selenium, a nutrient element that has a massive function in biological systems, is one of the interesting compounds to integrate with antibacterial agents. Selenium is an essential trace element in the diet, required for maintenance of health and growth 6 . Given that the least toxic form of Se is elemental Se 7 , its nano form has attracted significant attention 8 . In recent years, several studies have pointed out the ability of selenium nanoparticles to exhibit anticancer 9 , antioxidant 10 , antibacterial and anti-biofilm 11 properties. So far, remarkable antimicrobial activity of these nanoparticles have been evidenced against pathogenic bacteria, fungi and yeasts 12 , 13 , 14 .
Lysozyme is a biomolecule that has been widely distributed in humans, vertebrates, plants, bacteria and phages, which plays an important defensive role in the innate immune system. It is known as an antibacterial enzyme that accelerates hydrolyzes of β1,4 glucosidic linkages between N-acetylglucosamine (NAG) and N-acetylmuramic acid (NAM) in peptidoglycan of the cell wall, especially in gram-positive bacteria 15 . Furthermore, there are evidences that lysozyme could be an effective agent for killing human immunodeficiency virus (HIV) 16 , 17 . Chicken egg white lysozyme (HEWL), as a highly abundant small globular protein, has a vast industrial use as safe food preservative 18 , 19 . HEWL has also been widely considered as a model to study its interaction with various nanomaterials such as gold nanorods 20 , nano diamonds 21 , silver 22 , ZnO 23 and polymeric nanoparticles 24 .
Considering the natural antimicrobial effects of lysozyme, as well as the promising antimicrobial potential of selenium nanoparticles, the present study has been carried out to integrate selenium nanoparticles with lysozyme, based on the hypothesis that presence of the nano and bio counterparts in form of a Nanohybrid system might induce promising synergistic effect. Herein, the antibacterial activity of pristine SeNPs and lysozyme, as well as their complex form, has been investigated on standard strains of S. aureus and E. coli . To the best of our knowledge, this is the first effort to study the possible synergistic antibacterial effect of selenium nanoparticles and lysozyme.
Results and Discussion
Characterization of se nanoparticles.
In this study, a facile wet chemical method was utilized for synthesis of monodisperse SeNPs by Ascorbic acid with biocompatibility and good reducing properties. Selenium oxyanion SeO 3 2 ) was formed in aqueous medium. When this solution reacted with ascorbic acid, selenium was reduced to elemental selenium (Se 0 ).
The characteristic absorption peak of the Se nanoparticles is shown in Fig. 1a . Appearance of a sharp peak at 265 nm as well as the intense orange color of the colloidal dispersion (Fig. 1a , the inset) shows formation of Se nanoparticles. Characterization of the purified samples by scanning electron microscopy depicted the spherical morphology of the nanoparticles (Fig. 1b ). Analysis of SEM by Image J software showed that size of the nanoparticles was 35.6 ± 7.5 nm.
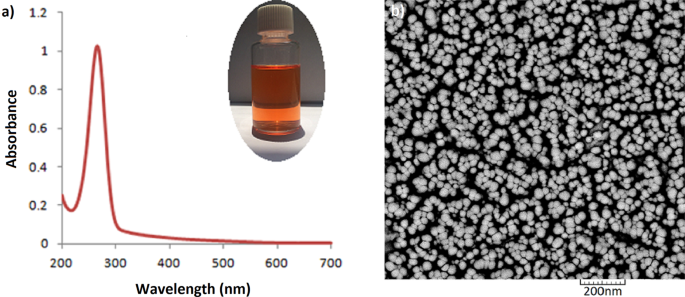
Absorption spectrum ( a ) and SEM image ( b ) of SeNPs. The inset shows color of the colloidal suspension.
Characterization of SeNPs-Lysozyme (the Nanohybrid system)
In order to monitor the interaction of nano and bio components, the dynamic light scattering (DLS) technique was used to compare hydrodynamic size of the purified SeNPs with their hybrid form. Figure 2 shows Z-average values of the SeNPs before and after interaction with lysozyme. Z-average size has become the accepted norm for presenting particle sizing results. Since the calculation of this parameter is mathematically stable, its result is insensitive to noise, making it a preferred DLS size parameter and a reliable measure of the average size of a particle size distribution. Z-average increases as the particle size increases. In the present study, this parameter increased from 71 to 84 nm for SeNPs, indicating the interaction between the nano and bio components (dimension of lysozyme is 4.5 × 3 × 3 nm). The polydispersity index (PDI) of the system also changed from 0.029 for the pristine SeNPs (implying narrow size distribution) to 0.30, giving an idea about notable changes in the nanoparticle size distribution upon interaction with lysozyme. Taking the zeta potential value of lysozyme into account (around +6.5 mV), protein adsorption onto Se nanoparticles induced a steady increase in zeta potential of nanoparticles. The zeta potential of untreated SeNPs was estimated to be −30.2 mV, which increased to −3.3 mV due to the adsorption of positively-charged lysozyme molecules onto the matrix of SeNPs (Fig. 2c,d ). This represents that electrostatic interactions have played a key role in formation of protein-loaded nanoparticles. It is worth to mention that zeta potential is an important indicator of the stability of colloidal suspensions. Therefore, the negative charge of the protein-loaded nanoparticles implies that the overall system exists in a stable condition 22 .
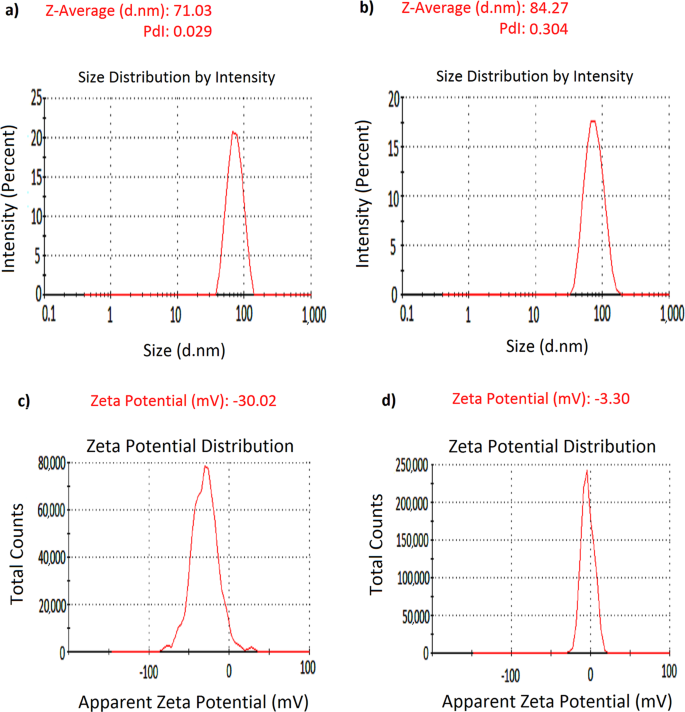
DLS measurements of SeNPs ( a ), and SeNPs-Lysozyme (100 µg/mL) ( b ), with Zeta potential of SeNPs ( c ), and Lysozyme-SeNPs ( d ), dispersed in 20 mM phosphate buffer, pH 6.2.
To monitor the interaction of Lysozyme with SeNPs, a fixed concentration of the nanoparticles was incubated with different concentrations of Lysozyme. Absorption spectra of SeNPs are depicted in Fig. 3a . The absorption intensity of the nanoparticles at their characteristic wavelength (265 nm) decreased upon increment of the biomolecule concentration. Nevertheless, the characteristic band has maintained its typical shape, showing that the nanoparticles have not experienced aggregation upon interaction with positively charged biomolecule. Appearance of the samples also confirmed the same (data not shown).
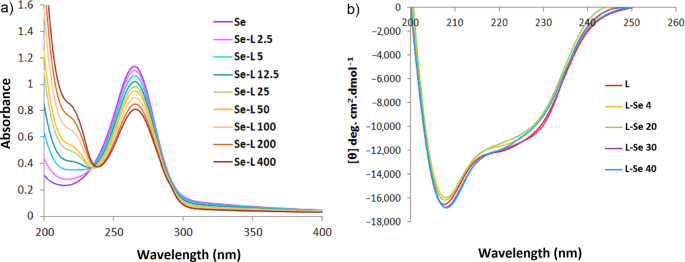
Absorption spectra of SeNPs upon interaction with different concentrations of lysozyme ( a ) and Far-UV CD spectra of lysozyme upon interaction with different concentrations of SeNPs ( b ), in 0.02 mM sodium phosphate buffer, pH 6.2. Se and L represent SeNPs and lysozyme, respectively.
Furthermore, to investigate possible effect of the nanoparticles on the biological component of the Nanohybrid system, Circular dichroism spectropolarimetry of lysozyme was monitored in the presence of different concentrations of the SeNPs. Based on a number of reports, in the context of developing Nanohybrid systems of interest (e.g. for targeted drug delivery purposes), it may be possible that upon interaction and surface adsorption of the biomolecules with nanoparticles some unfavorable conformational changes might occur in the structure of the bio component in the Nanohybrid system, leading to the altered function. On the other hand, there are cases with the nanoparticles in which, structural and functional properties have been improved from biophysical and biochemical aspects. Such facts bring the essence of conducting a series of fundamental studies into notice, before application of any nano-based system for vitro/vivo purposes 20 , 22 , 25 .
Figure 3b depicts Far-UV CD spectra and compares the conformation of native lysozyme upon interaction with various concentrations of SeNPs. Circular dichroism is a very helpful technique to monitor induced conformational changes of the macromolecules. A glance at Fig. 3b shows that the characteristic bands of α-helical structure at 208 and 222 nm have negligibly gained less negative value upon interaction with low concentrations of SeNPs (i.e. 4 and 20 µg.mL −1 ), indicating a small decrease in the helical content of lysozyme. Comparison of the CD spectra of lysozyme with higher concentration of SeNPs (40 µg.mL −1 ) with respect to 30 µg.mL −1 does not show any notable changes in the secondary structure of the biomolecule. Therefore, as an overall conclusion in this section, lysozyme retained its native structure in the vicinity of SeNPs. This could be a useful data since it could be anticipated that presence of SeNPs as the nano component of the Nanohybrid system may not induce unfavorable effect on the catalytic activity (i.e. antibacterial property) of lysozyme. CD spectrum of the nanohybrid samples after removal of excess protein also shows that the protein bound to the nanoparticles has maintained its native conformation (Fig. S1 ).
Antibacterial efficiency of the individual components against S. aureus
To obtain information about the effective range of inhibition of SeNPs and Lysozyme on the bacterial growth, different concentrations of each component was independently tested against S. aureus . Figure 4a shows the growth rate of S. aureus under treatment with different concentrations of SeNPs. After 20 hours of incubation, all concentrations were found to effectively reduce bacterial growth. The minimum inhibitory concentration was calculated to be 82 μg.mL −1 . In order to conduct further experiments, two concentrations of 5 and 10 μg.mL −1 of selenium nanoparticles were selected with significant effects (p = 0.000) with respect to the control group.
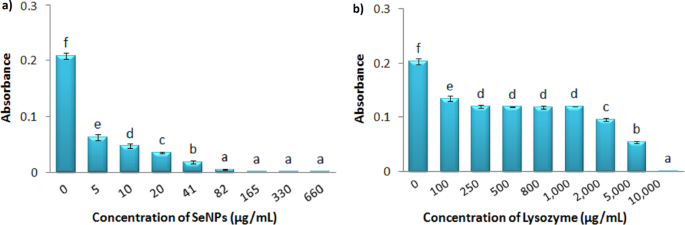
Growth Inhibition of S. aureus under treatment with different concentrations of SeNPs ( a ), and Lysozyme ( b ) after 20 hours. Absorbance was recorded at 630 nm. Each bar indicates mean ± standard deviations of three replications. Bars not labeled by the same letter represent statistical significance at P ≤ 0.05 using ANOVA followed by Tukey’s HSD test.
The same has been tested to monitor the growth rate of S. aureus under treatment with different concentrations of Lysozyme (Fig. 4b ). Analysis of data showed that within a wide range of concentration (100 μg.mL −1 to 1 mg.mL −1 ) of this enzyme there isn’t any notable change in the antibacterial activity of lysozyme. The MIC value in this experiment was estimated to be 10 mg.mL −1 . In order to investigate the antibacterial efficiency of the Nanohybrid system, the lowest concentration of lysozyme (100 μg.mL −1 ) was selected at a level of significance of p = 0.000 with respect to the control group.
Antibacterial efficiency of the individual components against E. coli
Figure 5a shows the growth of E . coli incubate with different concentrations of SeNPs. The gram-negative bacteria showed significant resistance to nanoparticles within a wide range of concentration. The same was observed when lysozyme was tested (Fig. 5b ). Within the specified ranges of concentration, neither the nanoparticles nor the protein could completely inhibit the growth of E. coli in this study. The highest inhibition was observed at 660 μg.mL −1 of SeNPs and 2 mg. mL −1 of Lysozyme (41%). To monitor the antibacterial efficiency of the Nanohybrid system, 330 μg.mL −1 of SeNPs (at a level of significance of p = 0.000 with respect to the control group) and 100, 2000, 5000 μg.mL −1 of Lysozyme (at a level of significance of p = 0.000 with respect to the control group) were selected for further experiments.
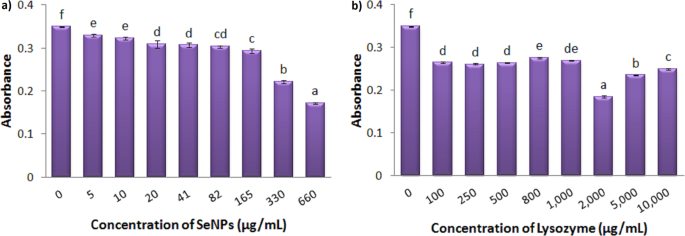
Growth Inhibition of E . coli under treatment with different concentrations of SeNPs ( a ) and Lysozyme ( b ) after 20 hours. Absorbance was recorded at 630 nm. Each bar indicates mean ± standard deviations of three replications. Bars not labeled by the same letter represent statistical significance at P ≤ 0.05 using ANOVA followed by Tukey’s HSD test.
Antibacterial efficiency of the nanohybrid system against S. aureus and E. coli
Figure 6a compares the growth of S. aureus in the presence of individual components (i.e. SeNPs and Lysozyme) as well as the hybrid nano system. The nanohybrid system with the lowest concentration of lysozyme (10 μg.mL −1 ) has no effect as compared to those of SeNPs controls. Nevertheless, with other low concentrations i.e. 50 and 100 μg.mL −1 of lysozyme, the nanohybrid system containing the lowest amount of the nano component have induced complete inhibition of S. aureus growth. Upon interaction of all three concentrations of SeNPs (1, 5, 10 μg.mL −1 ) with Lysozyme, the nanohybrid system has efficiently enhanced the antibacterial activity with respect to the protein alone. Therefore, SeNPs play an important role in inhibition of bacterial growth at very low concentration of protein. Another important feature of this hybrid nano system is that lysozyme has also played a vital role in antibacterial property of SeNPs. Although individual Lysozyme (100 μg.mL −1 ) is not remarkably found effective on inhibition of S. aureus (see Fig. 4b ), its presence in the Nanohybrid system containing 1 μg.mL −1 SeNPs has presented ideal synergistic effect and 100% inhibition; whereas the nanoparticle itself has not induced such effect at this concentration.
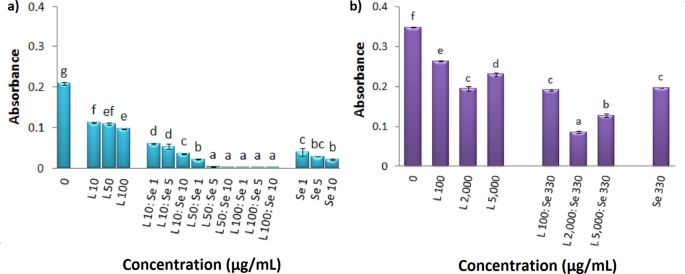
Antibacterial efficiency of Lysozyme, Nanohybrid system and SeNPs against S. aureus ( a ), E . coli ( b ). Absorbance was recorded at 630 nm. Se and L represent SeNPs and lysozyme, respectively. Each bar indicates mean ± standard deviations of three replications. Bars not labeled by the same letter represent statistical significance at P ≤ 0.05 using ANOVA followed by Tukey’s HSD test.
Hence, it can be concluded that although individual SeNPs have shown to efficiently reduce bacterial growth at low concentrations (MIC of 82 μg.mL −1 ) with respect to very high concentrations of Lysozyme (MIC of 10 mg.mL −1 ), presence of both nano and bio counterparts have induced vital interplay in the Nanohybrid system.
Figure 6b depicts antibacterial property of individual SeNPs and Lysozyme and their hybrid system against E. coli . The same trend has been observed in this case and interaction of nanoparticles with the biomolecule has led to improvement in the antibacterial efficiency with respect to the individual components against E. coli . The Nanohybrid system with 330 μg.mL −1 SeNPs and 2 mg.mL −1 Lysozyme is found to have presented the highest level of inhibition (74%).
Aging and stability study of the nanohybrid system
Prior to utilize any nanohybrid systems, it is very important to monitor the stability of the nanoparticles. Many times, upon ageing, the electrostatic interaction itself plays a key role in inducing severe decrease in the interparticle distance, thereby guiding the overall system into irreversible aggregation. Figure 7(a–d) compares stability of selenium nanoparticles as an intact and complex form with lysozyme. Interestingly, at much diluted sample of SeNPs (5 µg.mL −1 ), its complex form with lysozyme seems to provide more stability for the nanoparticles (Fig. 7a ). This is better observed when samples are aged up to 48 hours (see Fig. 7b ). Upon increment of SeNPs concentration (330 µg.mL −1 ), this is not observed and both the intact and complex form of SeNPs show good stability. It is worth mentioning that the nanohybrid system retains its appearance and the characteristic absorption band even after several weeks of storage under refrigerated condition (Fig. S2 ).
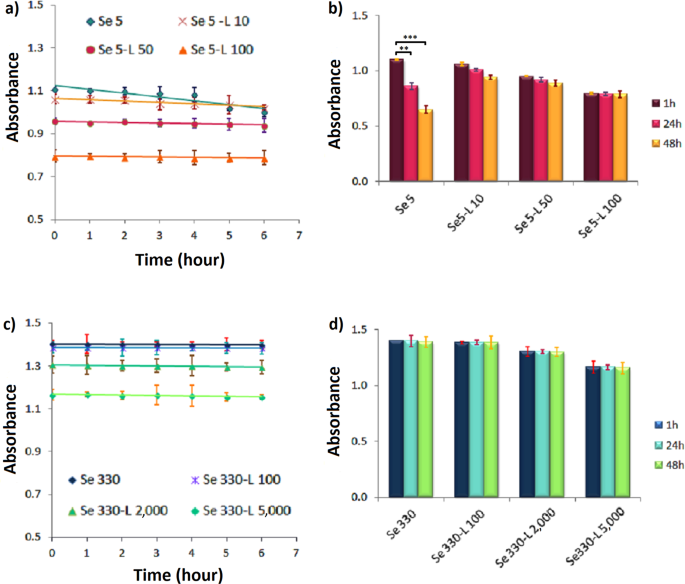
Aging of the pristine and protein-loaded form of SeNPs with two different concentrations of 5 ( a ) and 330 µg/mL ( c ) after 6 h ( a,c ), and 48 h ( b,d ) of incubation with the optimized concentrations of lysozyme. Absorbance was recorded at 265 nm for SeNPs. Se and L represent SeNPs and lysozyme, respectively.
A glance at the available reports shows that the UV-vis spectrum of SeNPs is in agreement with previous studies 26 , 27 , and the nanoparticles have been synthesized at a monodisperse state with spherical morphology 7 , 28 . The integration of different concentrations of nanoparticles with lysozyme did not induce any adverse effect on any of the components and both nanostructures and protein showed good stability and maintained native conformation, respectively. This is the first time that conformation of lysozyme has been monitored upon interaction with SeNPs.
Several studies have shown the antimicrobial activity of selenium nanoparticles which is consistent with our findings 29 , 30 . The difference in the available results toward growth inhibition may originate from the differences in the synthesis method, size, morphology, purity and concentration of SeNPs, as well as different bacterial strains or methods for evaluation of antibacterial activities.
There is no report to monitor the antibacterial activity of SeNPs in form of a Nanohybrid system with lysozyme to obtain synergistic effects. There are several studies which show improved antibacterial effects when lysozyme is used in combination with other nanomaterials. Ernest et al . reported a relative synergistic bactericidal effect between lysozyme and AgNPs compared to antibacterial properties of free lysozyme 31 . In a study conducted by Tripathy et al ., the MIC value for lysozyme–ZnO nanoparticle conjugate towards S. aureus and E . coli were evaluated to be 18 and 12 μg.mL −1 , respectively: while for bare lysozyme it was found to be 191 and 236 μg.mL −1 , respectively 32 . Abu Hamed et al ., observed the synergistic effect of lysozyme bound to aminated cellulose nanocrystals (AM-CMC) Am-CNC-lysozyme conjugates that inhibited E . coli growth at 650 μg.mL −1 33 . Moreover, Wu et al ., stated that the integration of lysozyme into chitosan nanoparticles (CS-Lys-NPs) increased the antibacterial activity of nanoparticles against E. coli, so that the MIC value of chitosan nanoparticles bound to lysozyme decreased from 5.8 to 5.5 mg.mL −1 34 . Yu et al . also constructed a hybrid coating on titanium implant containing lysozyme (10 mg.mL −1 ), chitosan, silver and hydroxyapatite 35 . Antimicrobial and cell culture tests showed that the Lys/CS/Ag/HAp hybrid coating not only boosted the antibacterial activity of titanium, but also revealed little toxicity to cell activity due to the low Ag content. The antibacterial efficiency of Lys/CS/Ag/HAp-Ti could increase to 95.28% and 98.2% against E. coli and S. aureus , respectively. Hence, the hybrid coating on Ti implant endowed this material with highly effective antibacterial activity and biocompatibility.
SeNPs with lower cytotoxic effects than those of AgNPs have attracted significant attention these days. Nevertheless, conclusive reports on the role of size, monodispersity, morphology, surface charge, etc. on their antibacterial efficiency are scarce. Moreover, there is not enough record to monitor and compare the possible synergistic effect of these nanoparticles in hybrid systems. A very recent study conducted by Jamroz et al ., reported incorporation of SeNPs and AgNPs into furcellaran-gelatin (FUR/GEL) films 36 . They observed that FUR/GEL films containing SeNPs showed greater antimicrobial activity against Escherichia coli, Staphylococcus aureus and MRSA than films containing AgNPs. Therefore, it can be assumed that SeNPs can be a promising candidate for the replacement of AgNPs in the upcoming antibacterial applications.
Results of the antibacterial tests on S. aureus and E . coli indicated that nanoparticles, lysozyme and also the hybrid system showed great inhibitory effect on the growth of Gram + bacteria as well as the growth of Gram- bacteria (at higher doses). Since lysozyme acts as a β-glucosidase enzyme to hydrolyze 1, 4-beta-linkages in the cell wall of some microorganisms, it can be concluded that lysozyme is an enzyme effective on gram + bacteria. Meanwhile, the lipopolysaccharide (LPS) layer in Gram- bacteria protects them against this protein 37 , 38 .
The mechanism of the cytotoxicity of SeNPs against bacteria has still remained unclear. Zeta potential of nanoparticles is important in interacting with bacteria. Many bacteria have a negative surface charge. Due to the additional layer of negatively charged lipopolysaccharides, Gram negative bacteria are more negatively charged than Gram positive bacteria 39 , 40 . Therefore, it is expected that strong repulsive forces exist between SeNPs and highly negative charged bacteria such as E . coli . For bacteria with a lower (or neutral) surface net charge, such as S. aureus , the interaction between SeNPs and bacteria can help nanoparticles to deposit on the membrane of the bacteria, which can have adverse effects on the cell division or bacterial survival 7 . Overall, difference in the cell wall composition and the surface characteristics of two types of bacteria contributes to the difference in the inhibitory effects between gram- and gram + bacteria. In this research, the negative charge of the hybrid system is reduced compared with nanoparticles and consequently this results in less electrostatic repellency when exposed to bacteria. This is probably one of the reasons that acknowledge the synergistic effect of the hybrid system.
This effort investigated the antibacterial activity of selenium nanoparticles in form of a nanohybrid system. SeNPs were incubated with Lysozyme and the antimicrobial property of the nanohybrid system was monitored against Escherichia coli and Staphylococcus aureus . The MIC value for SeNPs against Staphylococcus aureus was found to be 82 μg.mL −1 ; whereas very high concentrations of Lysozyme were required for 100% inhibition (10 mg.mL −1 ). Upon interaction of SeNPs with the biomolecule, both nano and bio counterparts induced vital interplay in the nanohybrid system with very low concentrations, leading to synergistic effect on the antibacterial property against both bacterial strains. Study of the aged samples of the nanohybrid system also revealed good stability of SeNPs both as the intact and complex form. Results of this effort encourage utilization of nanohybrid systems with synergistic antibacterial properties for a variety of applications in medicine, biomedicine, food safety and health care products. Nonetheless, more investigations are required to fully elucidate the mechanism and effects of cytotoxicity and antibacterial properties of such types of nanohybrid systems.
Chemicals and bacterial strains
Lysozyme from chicken egg white was purchased from Bio Basic. Sodium selenite, Sodium dihydrogen phosphate dehydrate, Disodium monohydrogen phosphate were procured from Merck. Ascorbic acid and Polysorbate 20 were purchased from Sigma. Muller-Hinton broth/agar were purchased from HiMedia. Escherichia coli (ATCC25922) and Staphylococcus aureus (ATCC25923), were obtained from the department of Bacteriology of Tarbiat Modares University, Iran. Ultrapure deionized water (DI) was used during the whole experiments. Glassware was thoroughly cleaned with dilute sulfo chromic acid/detergent solution and rinsed with DI.
Synthesis of selenium nanoparticles
SeNPs were prepared via a reduction of sodium selenite by ascorbic acid and stabilized by polysorbate 20. Briefly, 30 mg of Na 2 SeO 3 .5H 2 O was added to 90 mL of Milli-Q water. Ascorbic acid (10 mL, 56.7 mM) was added dropwise to sodium selenite solution with vigorous stirring.10 µL of polysorbate were added after each 2 ml of ascorbic acid. Selenium nanoparticles were formed after the addition of ascorbic acid. This can be visualized by a color change of the reactant solution from clear white to clear red. All solutions were made in a sterile environment by using a sterile cabinet and double distilled water. Selenium nanoparticles were then collected by centrifuging the solution at 12000 rpm. The pellet was resuspended in sterile double distilled water before use in bacteria experiments. Selenium contents of nanoparticles were determined using inductively coupled plasma optical emission spectroscopy (ICP-OES, model Vista-Pro from Varian).
SEM and UV–Vis characterization
Size, morphology and distribution of SeNPs were studied by scanning electron microscopy (SEM). Dilute sample was prepared and well dispersed by sonication. Samples were then dried and labelled with a gold monolayer (sputter coating), imaged by Qunta 200 electron microscope. SeNPs were also characterized by UV–Vis absorption spectrophotometer (PerkinElmer, Lambda 25). The spectra were recorded within 200–500 nm wavelength range.
Nanoparticle-protein interaction
SeNPs were mixed with different concentrations of protein in sodium phosphate buffer (20 mM, pH 6.2). Samples were then incubated at ambient temperature for 1 h prior to characterization. For analysis of protein conformation, a fixed concentration of lysozyme (200 μg.mL −1 in phosphate buffer 20 mM, pH 6.2) was mixed with various concentrations of SeNPs at ambient temperature. Longer incubation time did not affect the interaction. All experiments were performed with the as-prepared nanohybrid system.
Dynamic light scattering
Effects of protein concentration on the average hydrodynamic size of nanoparticles were monitored using dynamic light scattering. The solvent should be of high purity (MiliQ water), without large molar mass impurities (e.g. dust) to avoid “shadowing” of the signal for the scattering particles. Surface charge of the nanoparticles was determined using Malvern Zetasizer Nano-ZS instrument.
Circular dichroism spectropolarimetry
Circular dichroism (CD) measurements were carried out on a JASCO spectropolarimeter (J-715) to monitor possible changes that SeNPs might induce in lysozyme’s conformation. Far UV-CD region was scanned in the wavelength range of 200–250 nm. CD is reported in terms of mean residue ellipticity [θ] in deg.cm 2 .dmol −1 , as depicted in the following equation:
Where, θ is molar ellipticity, Mw is the lysozyme molecular weight, n is the number of amino acid residues, c is the lysozyme concentration in mg.mL −1 , and l is the light path length in centimeters. Data was smoothed and analysed by Jasco software, after subtracting the buffer and SeNPs contribution from the original protein and protein–SeNPs complex, respectively.
Antibacterial activity test of nanohybrid system
SeNPs, Lysozyme and nanohybrid system were tested for minimum inhibitory concentration (MIC) leading to the inhibition of bacterial growth by standard microdilution method (provided by the CLSI, 2018). MIC is the lowest concentration of an antimicrobial agent that prevents visible growth of a microorganism in an agar or broth dilution susceptibility test. A 96-well microtiter plate dilution was applied to determine the MIC. Briefly, 50 µL of twice the desired final concentration of antimicrobial agent was added to each well containing 50 µL Muller-Hinton broth. The inoculums were prepared by using direct colony suspension method by making a direct saline suspension of isolated colonies selected from a 24 h agar plate and then adjust the suspension to achieve a turbidity equivalent to a 0.5 McFarland standard. A total of 10 µL of activated culture of each tested strain (about 5 × 10 6 CFU/mL) was added. After 20 h of incubation at 37 °C, bacterial growth inhibition was determined by monitoring the optical density at 630 nm by ELX 808 microplate reader (Biotek Instruments, Winooski VT, USA). Absorbance at 630 nm showed the bacterial growth. The MIC value was recorded as the lowest concentration of the sample inhibiting the visible growth of microorganisms. A blank control without the sample was used for the control
Statistical analysis
All quantitative tests were carried out over three times and then mean values with standard deviations were calculated. Statistical analyses were performed using SPSS 16 software. Data were collected, and the significant differences were assessed with the probability associated with one-way ANOVA tests. One-way ANOVA followed by Tukey’s multiple-comparison post hoc tests were exploited for the comparison of three or more groups. The significance level ( p ) was considered at 0.05.
Data availability
All raw and analyzed data as well as the materials are available in this study.
Change history
25 november 2020.
Editor’s Note: Authorship of this article is currently under dispute. Appropriate editorial action will be taken once this matter is resolved.
30 November 2021
Editor's Note: Based on the explanation provided by the authors and their institution, the Editors concluded that no further editorial action is needed.
Vimbela, G. V., Ngo, S. M., Fraze, C., Yang, L. & Stout, D. A. Antibacterial properties and toxicity from metallic nanomaterials. International Journal of Nanomedicine , https://doi.org/10.2147/IJN.S134526 (2017).
Morens, D. M., Folkers, G. K. & Fauci, A. S. Emerging infections: a perpetual challenge. Lancet Infect. Dis. 8 , 710–719 (2008).
Article Google Scholar
Moghimi, S. M., Hunter, A. C. & Murray, J. C. Nanomedicine: current status and future prospects. FASEB J. 19 , 311–330 (2005).
Article CAS Google Scholar
Alpaslan, E., Geilich, B. M., Yazici, H. & Webster, T. J. pH-controlled cerium oxide nanoparticle inhibition of both gram-positive and gram-negative bacteria growth. Sci. Rep. 7 , 45859 (2017).
Article ADS CAS Google Scholar
Beyth, N., Houri-Haddad, Y., Domb, A., Khan, W. & Hazan, R. Alternative antimicrobial approach: Nano-antimicrobial materials. Evidence-based Complementary and Alternative Medicine , https://doi.org/10.1155/2015/246012 (2015).
Skalickova, S. et al . Selenium nanoparticles as a nutritional supplement. Nutrition 33 (2017).
Tran, P. A. et al . Low cytotoxic trace element selenium nanoparticles and their differential antimicrobial properties against S. aureus and E. coli . Nanotechnology 27 (2015).
Hosnedlova, B. et al . IJN-157541-nano-selenium-and-its-nanomedicine-applications–a-critical. Int. J. Nanomedicine Dovepress 13 , 2107–2128 (2018).
Yu, B., Zhang, Y., Zheng, W., Fan, C. & Chen, T. Positive surface charge enhances selective cellular uptake and anticancer efficacy of selenium nanoparticles. Inorg. Chem. 51 , 8956–8963 (2012).
Forootanfar, H. et al . Antioxidant and cytotoxic effect of biologically synthesized selenium nanoparticles in comparison to selenium dioxide. J. Trace Elem. Med. Biol. 28 , 75–79 (2014).
Shakibaie, M., Forootanfar, H., Golkari, Y., Mohammadi-Khorsand, T. & Shakibaie, M. R. Anti-biofilm activity of biogenic selenium nanoparticles and selenium dioxide against clinical isolates of Staphylococcus aureus, Pseudomonas aeruginosa, and Proteus mirabilis. J. Trace Elem. Med. Biol. 29 , 235–241 (2015).
Hariharan, H., Al-Dhabi, N. A., Karuppiah, P. & Rajaram, S. K. Microbial synthesis of selenium nanocomposite using Saccharomyces cerevisiae and its antimicrobial activity against pathogens causing nosocomial infection. Chalcogenide Lett 9 , 509–515 (2012).
CAS Google Scholar
Shahverdi, A. R. et al . Antifungal activity of biogenic selenium nanoparticles. World Appl. Sci. J. 10 , 918–922 (2010).
Beheshti, N. et al . Efficacy of biogenic selenium nanoparticles against Leishmania major: in vitro and in vivo studies. J. Trace Elem. Med. Biol. 27 , 203–207 (2013).
Cegielska-Radziejewska, R., Lesnierowski, G. & Kijowski, J. Antibacterial activity of hen egg white lysozyme modified by thermochemical technique. Eur. Food Res. Technol. 228 , 841–845 (2009).
Lee-Huang, S. et al . Structural and functional modeling of human lysozyme reveals a unique nonapeptide, HL9, with anti-HIV activity. Biochemistry 44 , 4648–4655 (2005).
Behbahani, M., Nosrati, M. & Mohabatkar, H. Inhibition of human immunodeficiency type 1 virus (HIV-1) life cycle by different egg white lysozymes. Appl. Biochem. Biotechnol. 185 , 786–798 (2018).
Zimoch-Korzycka, A. & Jarmoluk, A. The use of chitosan, lysozyme, and the nano-silver as antimicrobial ingredients of edible protective hydrosols applied into the surface of meat. J. Food Sci. Technol. 52 , 5996–6002 (2015).
Hughey, V. L. & Johnson, E. A. Antimicrobial activity of lysozyme against bacteria involved in food spoilage and food-borne disease. Appl. Environ. Microbiol. 53 , 2165–70 (1987).
Tohidi Moghadam, T., Ranjbar, B. & Khajeh, K. Conformation and activity of lysozyme on binding to two types of gold nanorods: A comparative study. Int. J. Biol. Macromol . 51 (2012).
Nguyen, T.-T.-B., Chang, H.-C. & Wu, V. W.-K. Adsorption and hydrolytic activity of lysozyme on diamond nanocrystallites. Diam. Relat. Mater. 16 , 872–876 (2007).
Ashrafpour, S. & Tohidi Moghadam, T. Interaction of silver nanoparticles with Lysozyme: Functional and structural investigations. Surfaces and Interfaces , https://doi.org/10.1016/j.surfin.2017.09.010 (2017).
Chakraborti, S. et al . Structure and activity of lysozyme on binding to ZnO nanoparticles. Langmuir 26 , 3506–3513 (2009).
Yu, E. et al . Poly (N-isopropylacrylamide)-gated Fe3O4/SiO2 core shell nanoparticles with expanded mesoporous structures for the temperature triggered release of lysozyme. Colloids Surfaces B Biointerfaces 135 , 652–660 (2015).
Tohidi Moghadam, T. et al . Interaction of lysozyme with gold nanorods: conformation and activity investigations. Int. J. Biol. Macromol. 49 , 629–636 (2011).
Gangadoo, S., Stanley, D., Hughes, R. J., Moore, R. J. & Chapman, J. The synthesis and characterisation of highly stable and reproducible selenium nanoparticles. Inorg. Nano-Metal Chem. 47 , 1568–1576 (2017).
Selim, N. A., Radwan, N. L., Youssef, S. F., Eldin, T. A. S. & Elwafa, S. A. Effect of inclusion inorganic, organic or nano selenium forms in broiler diets on: 2-Physiological, immunological and toxicity statuses of broiler chicks. Int. J. Poult. Sci. 14 , 144 (2015).
Cremonini, E. et al . Biogenic selenium nanoparticles: characterization, antimicrobial activity and effects on human dendritic cells and fibroblasts. Microb. Biotechnol , https://doi.org/10.1111/1751-7915.12374 (2016).
Chudobova, D. et al . Comparison of the effects of silver phosphate and selenium nanoparticles on Staphylococcus aureus growth reveals potential for selenium particles to prevent infection. FEMS Microbiol. Lett. 351 , 195–201 (2014).
Nguyen, T. H. D., Vardhanabhuti, B., Lin, M. & Mustapha, A. Antibacterial properties of selenium nanoparticles and their toxicity to Caco-2 cells. Food Control , https://doi.org/10.1016/j.foodcont.2017.01.018 (2017).
Ernest, V., Gajalakshmi, S., Mukherjee, A. & Chandrasekaran, N. Enhanced activity of lysozyme-AgNP conjugate with synergic antibacterial effect without damaging the catalytic site of lysozyme. Artif. cells, nanomedicine, Biotechnol. 42 , 336–343 (2014).
Tripathy, N., Ahmad, R., Bang, S. H., Min, J. & Hahn, Y.-B. Tailored lysozyme–ZnO nanoparticle conjugates as nanoantibiotics. Chem. Commun. 50 , 9298–9301 (2014).
Abouhmad, A., Dishisha, T., Amin, M. A. & Hatti-Kaul, R. Immobilization to positively charged cellulose nanocrystals enhances the antibacterial activity and stability of hen egg white and T4 lysozyme. Biomacromolecules 18 , 1600–1608 (2017).
Wu, T. et al . Integration of lysozyme into chitosan nanoparticles for improving antibacterial activity. Carbohydr. Polym. 155 , 192–200 (2017).
Yu, W.-Z. et al . Synergistic antibacterial activity of multi components in lysozyme/chitosan/siler/hydroxyapatite hybrid coating. Mater. Des. 139 , 351–362 (2018).
Jamróz, E. et al . Development and characterisation of furcellaran-gelatin films containing SeNPs and AgNPs that have antimicrobial activity. Food Hydrocoll. 83 , 9–16 (2018).
Masschalck, B., Van Houdt, R., Van Haver, E. G. R. & Michiels, C. W. Inactivation of gram-negative bacteria by lysozyme, denatured lysozyme, and lysozyme-derived peptides under high hydrostatic pressure. Appl. Environ. Microbiol. 67 , 339–344 (2001).
Gill, A. O. & Holley, R. A. Interactive inhibition of meat spoilage and pathogenic bacteria by lysozyme, nisin and EDTA in the presence of nitrite and sodium chloride at 24 C. Int. J. Food Microbiol. 80 , 251–259 (2003).
Domingues, M. M., Santiago, P. S., Castanho, M. A. R. B. & Santos, N. C. What can light scattering spectroscopy do for membrane‐active peptide studies? J. Pept. Sci. an Off. Publ. Eur. Pept. Soc. 14 , 394–400 (2008).
Alves, C. S. et al . Escherichia coli cell surface perturbation and disruption induced by antimicrobial peptides BP100 and pepR. J. Biol. Chem. 285 , 27536–27544 (2010).
Download references
Acknowledgements
The authors would like to acknowledge Tarbiat Modars Univrsity and Isalamic Azad University for providing characterization facilities. The materials for conducting this project are self-funded. The universities have provided facilities for characterization of the Nanohybrid system.
Author information
Authors and affiliations.
Department of Biology, Science and Research Branch, Islamic Azad University, Tehran, Iran
Mahsa Vahdati
Department of Nanobiotechnology, Faculty of Biological Sciences, Tarbiat Modares University, Tehran, Iran
Tahereh Tohidi Moghadam
You can also search for this author in PubMed Google Scholar
Contributions
Mahsa Vahdati conducted the experiments, analyzed data and wrote some parts of the manuscript. Tahereh Tohidi Moghadam designed the experiments, supervised the project and wrote some parts of the manuscript.
Corresponding author
Correspondence to Tahereh Tohidi Moghadam .
Ethics declarations
Competing interests.
The authors declare no competing interests.
Additional information
Publisher’s note Springer Nature remains neutral with regard to jurisdictional claims in published maps and institutional affiliations.
Supplementary information
Supplementary figures s1 and s2., rights and permissions.
Open Access This article is licensed under a Creative Commons Attribution 4.0 International License, which permits use, sharing, adaptation, distribution and reproduction in any medium or format, as long as you give appropriate credit to the original author(s) and the source, provide a link to the Creative Commons license, and indicate if changes were made. The images or other third party material in this article are included in the article’s Creative Commons license, unless indicated otherwise in a credit line to the material. If material is not included in the article’s Creative Commons license and your intended use is not permitted by statutory regulation or exceeds the permitted use, you will need to obtain permission directly from the copyright holder. To view a copy of this license, visit http://creativecommons.org/licenses/by/4.0/ .
Reprints and permissions
About this article
Cite this article.
Vahdati, M., Tohidi Moghadam, T. Synthesis and Characterization of Selenium Nanoparticles-Lysozyme Nanohybrid System with Synergistic Antibacterial Properties. Sci Rep 10 , 510 (2020). https://doi.org/10.1038/s41598-019-57333-7
Download citation
Received : 23 July 2019
Accepted : 28 December 2019
Published : 16 January 2020
DOI : https://doi.org/10.1038/s41598-019-57333-7
Share this article
Anyone you share the following link with will be able to read this content:
Sorry, a shareable link is not currently available for this article.
Provided by the Springer Nature SharedIt content-sharing initiative
This article is cited by
A comparative study on green synthesis and characterization of mn doped zno nanocomposite for antibacterial and photocatalytic applications.
- Murtaza Hasan
- Mansour Ghorbanpour
Scientific Reports (2024)
Enhanced Bactericidal Effects and Drug Delivery with Gentamicin-Conjugated Nanoparticles
- I. D. Athauda
- M. G. Shetty
- K. S. Babitha
Journal of Cluster Science (2024)
A Critical Review on Nano-selenium Based Materials: Synthesis, Biomedicine Applications and Biocompatibility Assessment
- S. Karthick Raja Namasivayam
- S. Krithika Shree
Journal of Inorganic and Organometallic Polymers and Materials (2024)
Synthesis and Characterization of Bimetallic Platinum/Selenium (Pt/Se) Nanoparticles for Synergistic Antibacterial Activity
- Kandasamy Saravanakumar
- Anbazhagan Sathiyaseelan
- Myeong-Hyeon Wang
BioNanoScience (2024)
Synthesis and characterization of Se doped Fe3O4 nanoparticles for catalytic and biological properties
- Mohammad Reza Ahghari
- Zeinab Amiri-khamakani
Scientific Reports (2023)
By submitting a comment you agree to abide by our Terms and Community Guidelines . If you find something abusive or that does not comply with our terms or guidelines please flag it as inappropriate.
Quick links
- Explore articles by subject
- Guide to authors
- Editorial policies
Sign up for the Nature Briefing newsletter — what matters in science, free to your inbox daily.


Page load error
We’re sorry..
The site has encountered an unexpected error and this page is currently unavailable.
Our engineers have been automatically notified of this error and will work to resolve it as soon as possible.
Please try again later, or return to the home page and start again.
If the problem persists, please contact our Technical Support team for assistance.

An official website of the United States government
The .gov means it’s official. Federal government websites often end in .gov or .mil. Before sharing sensitive information, make sure you’re on a federal government site.
The site is secure. The https:// ensures that you are connecting to the official website and that any information you provide is encrypted and transmitted securely.
- Publications
- Account settings
Preview improvements coming to the PMC website in October 2024. Learn More or Try it out now .
- Advanced Search
- Journal List
- Int J Mol Sci

Silver Nanoparticles: Synthesis, Characterization, Properties, Applications, and Therapeutic Approaches
Xi-feng zhang.
1 College of Biological and Pharmaceutical Engineering, Wuhan Polytechnic University, Wuhan 430023, China; moc.361@5649fxgnahz (X.-F.Z.); moc.621@l_ougihz (Z.-G.L.)
Zhi-Guo Liu
2 Key Laboratory of Animal Reproduction and Germplasm Enhancement in Universities of Shandong, College of Animal Science and Technology, Qingdao Agricultural University, Qingdao 266109, China; moc.621@724iewnehs
Sangiliyandi Gurunathan
3 Department of Stem Cell and Regenerative Biotechnology, Konkuk University, Seoul 143-701, Korea
Recent advances in nanoscience and nanotechnology radically changed the way we diagnose, treat, and prevent various diseases in all aspects of human life. Silver nanoparticles (AgNPs) are one of the most vital and fascinating nanomaterials among several metallic nanoparticles that are involved in biomedical applications. AgNPs play an important role in nanoscience and nanotechnology, particularly in nanomedicine. Although several noble metals have been used for various purposes, AgNPs have been focused on potential applications in cancer diagnosis and therapy. In this review, we discuss the synthesis of AgNPs using physical, chemical, and biological methods. We also discuss the properties of AgNPs and methods for their characterization. More importantly, we extensively discuss the multifunctional bio-applications of AgNPs; for example, as antibacterial, antifungal, antiviral, anti-inflammatory, anti-angiogenic, and anti-cancer agents, and the mechanism of the anti-cancer activity of AgNPs. In addition, we discuss therapeutic approaches and challenges for cancer therapy using AgNPs. Finally, we conclude by discussing the future perspective of AgNPs.
1. Introduction
Silver nanoparticles (AgNPs) are increasingly used in various fields, including medical, food, health care, consumer, and industrial purposes, due to their unique physical and chemical properties. These include optical, electrical, and thermal, high electrical conductivity, and biological properties [ 1 , 2 , 3 ]. Due to their peculiar properties, they have been used for several applications, including as antibacterial agents, in industrial, household, and healthcare-related products, in consumer products, medical device coatings, optical sensors, and cosmetics, in the pharmaceutical industry, the food industry, in diagnostics, orthopedics, drug delivery, as anticancer agents, and have ultimately enhanced the tumor-killing effects of anticancer drugs [ 4 ]. Recently, AgNPs have been frequently used in many textiles, keyboards, wound dressings, and biomedical devices [ 2 , 5 , 6 ]. Nanosized metallic particles are unique and can considerably change physical, chemical, and biological properties due to their surface-to-volume ratio; therefore, these nanoparticles have been exploited for various purposes [ 7 , 8 ]. In order to fulfill the requirement of AgNPs, various methods have been adopted for synthesis. Generally, conventional physical and chemical methods seem to be very expensive and hazardous [ 1 , 9 ]. Interestingly, biologically-prepared AgNPs show high yield, solubility, and high stability [ 1 ]. Among several synthetic methods for AgNPs, biological methods seem to be simple, rapid, non-toxic, dependable, and green approaches that can produce well-defined size and morphology under optimized conditions for translational research. In the end, a green chemistry approach for the synthesis of AgNPs shows much promise.
After synthesis, precise particle characterization is necessary, because the physicochemical properties of a particle could have a significant impact on their biological properties. In order to address the safety issue to use the full potential of any nano material in the purpose of human welfare, in nanomedicines, or in the health care industry, etc., it is necessary to characterize the prepared nanoparticles before application [ 10 , 11 ]. The characteristic feature of nanomaterials, such as size, shape, size distribution, surface area, shape, solubility, aggregation, etc. need to be evaluated before assessing toxicity or biocompatibility [ 12 ]. To evaluate the synthesized nanomaterials, many analytical techniques have been used, including ultraviolet visible spectroscopy (UV-vis spectroscopy), X-ray diffractometry (XRD), Fourier transform infrared spectroscopy (FTIR), X-ray photoelectron spectroscopy (XPS), dynamic light scattering (DLS), scanning electron microscopy (SEM), transmission electron microscopy (TEM), atomic force microscopy (AFM), and so on [ 13 , 14 ].
The biological activity of AgNPs depends on factors including surface chemistry, size, size distribution, shape, particle morphology, particle composition, coating/capping, agglomeration, and dissolution rate, particle reactivity in solution, efficiency of ion release, and cell type, and the type of reducing agents used for the synthesis of AgNPs are a crucial factor for the determination of cytotoxicity [ 15 ]. The physicochemical properties of nanoparticles enhance the bioavailability of therapeutic agents after both systemic and local administration [ 16 , 17 ] and other hand it can affect cellular uptake, biological distribution, penetration into biological barriers, and resultant therapeutic effects [ 18 , 19 ]. Therefore, the development of AgNPs with controlled structures that are uniform in size, morphology, and functionality are essential for various biomedical applications [ 20 , 21 , 22 , 23 , 24 ].
Cancer is a complex, multifactorial disease which has the characteristic feature of the uncontrolled growth and spread of abnormal cells caused by several factors, including a combination of genetic, external, internal, and environmental factors [ 25 ], and it is treated by various treatments including chemotherapy, hormone therapy, surgery, radiation, immune therapy, and targeted therapy [ 25 ]. Therefore, the challenge is to identify effective, cost-effective, and sensitive lead molecules that have cell-targeted specificity and increase the sensitivity. Recently, AgNPs have been shown much interest because of their therapeutic applications in cancer as anticancer agents, in diagnostics, and in probing. Taken literature into consideration, in this review we focused on recent developments in synthesis, characterization, properties, and bio-applications mainly on the antibacterial, antifungal, antiviral, anti-inflammatory, anti-cancer and anti-angiogenic properties of AgNPs in a single platform. This review also emphasizes mechanism of anticancer activity, therapeutic approaches and the challenges and limitations of nanoparticles in cancer therapy. Finally, this review ends with conclusion and the future perspective of AgNPs.
2. Synthesis of AgNPs
2.1. synthesis of agnps using physical and chemical methods.
Generally, the synthesis of nanoparticles has been carried out using three different approaches, including physical, chemical, and biological methods. In physical methods, nanoparticles are prepared by evaporation-condensation using a tube furnace at atmospheric pressure [ 26 , 27 , 28 , 29 ]. Conventional physical methods including spark discharging and pyrolysis were used for the synthesis of AgNPs [ 30 , 31 ]. The advantages of physical methods are speed, radiation used as reducing agents, and no hazardous chemicals involved, but the downsides are low yield and high energy consumption, solvent contamination, and lack of uniform distribution [ 32 , 33 , 34 , 35 , 36 ].
Chemical methods use water or organic solvents to prepare the silver nanoparticles [ 37 , 38 ]. This process usually employs three main components, such as metal precursors, reducing agents, and stabilizing/capping agents. Basically, the reduction of silver salts involves two stages (1) nucleation; and (2) subsequent growth. In general, silver nanomaterials can be obtained by two methods, classified as “top-down” and “bottom-up” [ 39 ]. The “top-down” method is the mechanical grinding of bulk metals with subsequent stabilization using colloidal protecting agents [ 40 , 41 ]. The “bottom-up” methods include chemical reduction, electrochemical methods, and sono-decomposition. The major advantage of chemical methods is high yield, contrary to physical methods, which have low yield. The above-mentioned methods are extremely expensive. Additionally, the materials used for AgNPs synthesis, such as citrate, borohydride, thio-glycerol, and 2-mercaptoethanol are toxic and hazardous [ 41 ]. Apart from these disadvantages, the manufactured particles are not of expected purity, as their surfaces were found to be sedimented with chemicals. It is also very difficult to prepare AgNPs with a well-defined size, requiring a further step for the prevention of particle aggregation [ 42 ]. In addition, during the synthesis process, too many toxic and hazardous byproducts are excised out. Chemical methods make use of techniques such as cryochemical synthesis [ 43 ], laser ablation [ 44 ], lithography [ 45 ], electrochemical reduction [ 46 ], laser irradiation [ 47 ], sono-decomposition [ 48 ], thermal decomposition [ 49 ], and chemical reduction [ 50 ]. The advantage of the chemical synthesis of nanoparticles are the ease of production, low cost, and high yield; however, the use of chemical reducing agents are harmful to living organisms [ 13 ]. Recently, Abbasi et al. explained a detailed account of synthesis methods, properties, and bio-application of AgNPs [ 51 ].
2.2. Green Chemistry Approach for the Synthesis of AgNPs
To overcome the shortcomings of chemical methods, biological methods have emerged as viable options. Recently, biologically-mediated synthesis of nanoparticles have been shown to be simple, cost effective, dependable, and environmentally friendly approaches and much attention has been given to the high yield production of AgNPs of defined size using various biological systems including bacteria, fungi, plant extracts, and small biomolecules like vitamins and amino acids as an alternative method to chemical methods—not only for AgNPs, but also for the synthesis of several other nanoparticles, such as gold and graphene [ 9 , 52 , 53 , 54 , 55 , 56 ]. Bio-sorption of metals by Gram-negative and Gram-positive bacteria provided an indication for the synthesis of nanoparticles before the flourishing of this biological method; however, the synthesized nanomaterials were as aggregates not nanoparticles [ 57 ]. Several studies reported the synthesis of AgNPs using green, cost effective, and biocompatible methods without the use of toxic chemicals in biological methods. In this green chemistry approach, several bacteria, including Pseudomonas stutzeri AG259 [ 58 ], Lactobacillus strains [ 59 ], Bacillus licheniformis [ 55 ]; Escherichia coli ( E. coli ) [ 9 ], Brevibacterium casei [ 60 ], fungi including Fusarium oxysporum [ 61 ], Ganoderma neo-japonicum Imazeki [ 62 ], plant extracts such as Allophylus cobbe [ 52 ], Artemisia princeps [ 63 ], and Typha angustifolia [ 64 ] were utilized. In addition to these, several biomolecules, such as biopolymers [ 65 ], starch [ 66 ], fibrinolytic enzyme [ 39 ], and amino acids [ 67 ] were used. The biological synthesis of nanoparticles depends on three factors, including (a) the solvent; (b) the reducing agent; and (c) the non-toxic material. The major advantage of biological methods is the availability of amino acids, proteins, or secondary metabolites present in the synthesis process, the elimination of the extra step required for the prevention of particle aggregation, and the use of biological molecules for the synthesis of AgNPs is eco-friendly and pollution-free. Biological methods seem to provide controlled particle size and shape, which is an important factor for various biomedical applications [ 68 ]. Using bacterial protein or plant extracts as reducing agents, we can control the shape, size, and monodispersity of the nanoparticles [ 9 ]. The other advantages of biological methods are the availability of a vast array of biological resources, a decreased time requirement, high density, stability, and the ready solubility of prepared nanoparticles in water [ 69 ].
The biological activity of AgNPs depends on the morphology and structure of AgNPs, controlled by size and shape of the particles [ 70 , 71 ]. As far as size and shape are concerned, smaller size and truncated-triangular nanoparticles seem to be more effective and have superior properties. Although many studies successfully synthesized AgNPs with different shape and size ranges, they still have certain limitations. To achieve control over morphology and structure, an excess of strong reducing agent such as sodium borohydride (NaBH 4 ) was used for the synthesis of monodisperse and uniform-sized silver colloids [ 72 ]. Compared to chemical methods, biological methods allow for more ease in the control of shape, size, and distribution of the produced nanoparticles by optimization of the synthesis methods, including the amount of precursors, temperature, pH, and the amount of reducing and stabilizing factors [ 9 , 73 ].

3. Characterization
The physicochemical properties of nanoparticles are important for their behavior, bio-distribution, safety, and efficacy. Therefore, characterization of AgNPs is important in order to evaluate the functional aspects of the synthesized particles. Characterization is performed using a variety of analytical techniques, including UV-vis spectroscopy, X-ray diffractometry (XRD), Fourier transform infrared spectroscopy (FTIR), X-ray photoelectron spectroscopy (XPS), dynamic light scattering (DLS), scanning electron microscopy (SEM), transmission electron microscopy (TEM), and atomic force microscopy (AFM). Several qualified books and reviews have presented the principles and usage of various kinds of analytical techniques for the characterization of AgNPs; however, the basics of the important techniques used for the characterization of AgNPs are detailed below for ease of understanding. For example, characterization of AgNPs using various analytical techniques prepared from culture supernatant of Bacillus species was given in Figure 1 .

Characterization of silver nanoparticles (AgNPs) prepared from Bacillus species using various analytical techniques. ( A ) Characterization of AgNPs by X-diffraction spectra of AgNPs; ( B ) Fourier transform infrared spectra of AgNPs; ( C ) Measurement of size distribution of AgNPs by dynamic light scattering; ( D ) Scanning electron microscopy images of AgNPs; ( E ). Transmission electron microscopy images of AgNPs.
3.1. UV-Visible Spectroscopy
UV-vis spectroscopy is a very useful and reliable technique for the primary characterization of synthesized nanoparticles which is also used to monitor the synthesis and stability of AgNPs [ 74 ]. AgNPs have unique optical properties which make them strongly interact with specific wavelengths of light [ 75 ]. In addition, UV-vis spectroscopy is fast, easy, simple, sensitive, selective for different types of NPs, needs only a short period time for measurement, and finally a calibration is not required for particle characterization of colloidal suspensions [ 76 , 77 , 78 ]. In AgNPs, the conduction band and valence band lie very close to each other in which electrons move freely. These free electrons give rise to a surface plasmon resonance (SPR) absorption band, occurring due to the collective oscillation of electrons of silver nano particles in resonance with the light wave [ 79 , 80 , 81 , 82 , 83 , 84 ]. The absorption of AgNPs depends on the particle size, dielectric medium, and chemical surroundings [ 81 , 82 , 83 , 84 , 85 ]. Observation of this peak—assigned to a surface plasmon—is well documented for various metal nanoparticles with sizes ranging from 2 to 100 nm [ 74 , 86 , 87 ]. The stability of AgNPs prepared from biological methods was observed for more than 12 months, and an SPR peak at the same wavelength using UV-vis spectroscopy was observed.
3.2. X-ray Diffraction (XRD)
X-ray diffraction (XRD) is a popular analytical technique which has been used for the analysis of both molecular and crystal structures [ 79 , 88 ], qualitative identification of various compounds [ 89 ], quantitative resolution of chemical species [ 90 ], measuring the degree of crystallinity [ 91 ], isomorphous substitutions [ 92 ], particle sizes [ 93 ], etc. When X-ray light reflects on any crystal, it leads to the formation of many diffraction patterns, and the patterns reflect the physico-chemical characteristics of the crystal structures. In a powder specimen, diffracted beams typically come from the sample and reflect its structural physico-chemical features. Thus, XRD can analyze the structural features of a wide range of materials, such as inorganic catalysts, superconductors, biomolecules, glasses, polymers, and so on [ 94 ]. Analysis of these materials largely depends on the formation of diffraction patterns. Each material has a unique diffraction beam which can define and identify it by comparing the diffracted beams with the reference database in the Joint Committee on Powder Diffraction Standards (JCPDS) library. The diffracted patterns also explain whether the sample materials are pure or contain impurities. Therefore, XRD has long been used to define and identify both bulk and nanomaterials, forensic specimens, industrial, and geochemical sample materials [ 95 , 96 , 97 , 98 , 99 , 100 , 101 , 102 , 103 , 104 ].
XRD is a primary technique for the identification of the crystalline nature at the atomic scale [ 10 , 14 , 88 , 105 ]. X-ray powder diffraction is a nondestructive technique with great potential for the characterization of both organic and inorganic crystalline materials [ 106 ]. This method has been used to measure phase identification, conduct quantitative analysis, and to determine structure imperfections in samples from various disciplines, such as geological, polymer, environmental, pharmaceutical, and forensic sciences. Recently, the applications have extended to the characterization of various nano-materials and their properties [ 106 ]. The working principle of X-ray diffraction is Bragg’s law [ 88 , 105 ]. Typically, XRD is based on the wide-angle elastic scattering of X-rays [ 10 , 14 , 88 , 107 , 108 , 109 ]. Although XRD has several merits, it has limited disadvantages, including difficulty in growing the crystals and the ability to get results pertaining only to single conformation/binding state [ 14 , 108 , 110 ]. Another drawback of XRD is the low intensity of diffracted X-rays compared to electron diffractions [ 110 , 111 ].
3.3. Dynamic Light Scattering
Physicochemical characterization of prepared nanomaterials is an important factor for the analysis of biological activities using radiation scattering techniques [ 10 , 14 , 112 ]. DLS can probe the size distribution of small particles a scale ranging from submicron down to one nanometer in solution or suspension [ 10 , 14 , 113 ]. Dynamic light scattering is a method that depends on the interaction of light with particles. This method can be used for the measurement of narrow particle size distributions, especially in the range of 2–500 nm [ 78 ]. Among the techniques for the characterization of nanoparticles, the most commonly used is DLS [ 114 , 115 , 116 ]. DLS measures the light scattered from a laser that passes through a colloid, and mostly relies on Rayleigh scattering from the suspended nanoparticles [ 117 ]. Next, the modulation of the scattered light intensity as a function of time is analyzed, and the hydrodynamic size of particles can be determined [ 118 , 119 , 120 ]. To evaluate the toxic potential of any nanomaterial, its characterization in solution is essential [ 11 ]. Therefore; DLS is mainly used to determine particle size and size distributions in aqueous or physiological solutions [ 12 ]. The size obtained from DLS is usually larger than TEM, which may be due to the influence of Brownian motion. DLS is a nondestructive method used to obtain the average diameter of nanoparticles dispersed in liquids. It has the special advantage of probing a large quantity of particles simultaneously; however, it has a number of sample-specific limitations [ 101 , 121 ].
3.4. Fourier Transform Infrared (FTIR) Spectroscopy
FTIR is able to provide accuracy, reproducibility, and also a favorable signal-to-noise ratio. By using FTIR spectroscopy, it becomes possible to detect small absorbance changes on the order of 10 −3 , which helps to perform difference spectroscopy, where one could distinguish the small absorption bands of functionally active residues from the large background absorption of the entire protein [ 122 , 123 , 124 , 125 , 126 , 127 , 128 ]. FTIR spectroscopy is frequently used to find out whether biomolecules are involved in the synthesis of nanoparticles, which is more pronounced in academic and industrial research [ 10 , 68 , 129 , 130 ]. Furthermore, FTIR has also been extended to the study of nano-scaled materials, such as confirmation of functional molecules covalently grafted onto silver, carbon nanotubes, graphene and gold nanoparticles, or interactions occurring between enzyme and substrate during the catalytic process [ 68 , 131 , 132 ]. Furthermore, it is a non-invasive technique. Finally, the advantages of FTIR spectrometers over dispersive ones are rapid data collection, strong signal, large signal-to-noise ratio, and less sample heat-up [ 133 ]. Recently, further advancement has been made in an FTIR method called attenuated total reflection (ATR)-FTIR spectroscopy [ 134 , 135 , 136 ]. Using ATR-FTIR, we can determine the chemical properties on the polymer surface, and sample preparation is easy compared to conventional FTIR [ 10 , 137 , 138 , 139 , 140 , 141 ]. Therefore, FTIR is a suitable, valuable, non-invasive, cost effective, and simple technique to identify the role of biological molecules in the reduction of silver nitrate to silver.
3.5. X-ray Photoelectron Spectroscopy (XPS)
XPS is a quantitative spectroscopic surface chemical analysis technique used to estimate empirical formulae [ 109 , 140 , 141 , 142 ]. XPS is also known as electron spectroscopy for chemical analysis (ESCA), [ 141 ]. XPS plays a unique role in giving access to qualitative, quantitative/semi-quantitative, and speciation information concerning the sensor surface [ 143 ]. XPS is performed under high vacuum conditions. X-ray irradiation of the nanomaterial leads to the emission of electrons, and the measurement of the kinetic energy and the number of electrons escaping from the surface of the nanomaterials gives XPS spectra [ 109 , 140 , 141 , 142 ]. The binding energy can be calculated from kinetic energy. Specific groups of starburst macromolecules such as P=S, aromatic rings, C–O, and C=O can be identified and characterized by XPS [ 144 ].
3.6. Scanning Electron Microscopy
Recently, the field of nanoscience and nanotechnology has provided a driving force in the development of various high-resolution microscopy techniques in order to learn more about nanomaterials using a beam of highly energetic electrons to probe objects on a very fine scale [ 145 , 146 , 147 ]. Among various electron microscopy techniques, SEM is a surface imaging method, fully capable of resolving different particle sizes, size distributions, nanomaterial shapes, and the surface morphology of the synthesized particles at the micro and nanoscales [ 10 , 117 , 137 , 148 , 149 ]. Using SEM, we can probe the morphology of particles and derive a histogram from the images by either by measuring and counting the particles manually, or by using specific software [ 117 ]. The combination of SEM with energy-dispersive X-ray spectroscopy (EDX) can be used to examine silver powder morphology and also conduct chemical composition analysis. The limitation of SEM is that it is not able to resolve the internal structure, but it can provide valuable information regarding the purity and the degree of particle aggregation. The modern high-resolution SEM is able to identify the morphology of nanoparticles below the level of 10 nm.
3.7. Transmission Electron Microscopy
TEM is a valuable, frequently used, and important technique for the characterization of nanomaterials, used to obtain quantitative measures of particle and/or grain size, size distribution, and morphology [ 10 , 109 , 150 ]. The magnification of TEM is mainly determined by the ratio of the distance between the objective lens and the specimen and the distance between objective lens and its image plane [ 150 ]. TEM has two advantages over SEM: it can provide better spatial resolution and the capability for additional analytical measurements [ 10 , 148 , 150 ]. The disadvantages include a required high vacuum, thin sample section [ 10 , 109 , 148 ], and the vital aspect of TEM is that sample preparation is time consuming. Therefore, sample preparation is extremely important in order to obtain the highest-quality images possible.
3.8. Atomic Force Microscopy
Generally, AFM is used to investigate the dispersion and aggregation of nanomaterials, in addition to their size, shape, sorption, and structure; three different scanning modes are available, including contact mode, non-contact mode, and intermittent sample contact mode [ 10 , 14 , 151 , 152 , 153 , 154 , 155 ]. AFM can also be used to characterize the interaction of nanomaterials with supported lipid bilayers in real time, which is not achievable with current electron microscopy (EM) techniques [ 113 ]. In addition, AFM does not require oxide-free, electrically conductive surfaces for measurement, does not cause appreciable damage to many types of native surfaces, and it can measure up to the sub-nanometer scale in aqueous fluids [ 156 , 157 ]. However, a major drawback is the overestimation of the lateral dimensions of the samples due to the size of the cantilever [ 158 , 159 ]. Therefore, we have to provide much attention to avoid erroneous measurements [ 160 ]. Furthermore, the choice of operating mode—no contact or contact—is a crucial factor in sample analysis [ 160 ].
3.9. Localized Surface Plasmon Resonance (LSPR)
LSPR is a coherent, collective spatial oscillation of the conduction electrons in a metallic nanoparticle, which can be directly excited by near-visible light. The localized surface plasmon resonance (LSPR) condition is defined by several factors, including the electronic properties of the nanoparticle, the size and shape of the particle, temperature, the dielectric environment, and so on. Small changes in the local dielectric environment cause the dysfunction of LSPR. The frequency of the LSPR spectral peak is very sensitive to the nanostructure environment through the local refractive index. Thereby, shifts of the LSPR frequency are widely used as a method for the detection of molecular interaction close to the surface of the nanoparticle [ 161 , 162 , 163 , 164 , 165 , 166 ]. In addition, the near-field enhancement has led to a very large variety of advances in many fundamental and applied areas of science, particularly for the determination of nanoparticle shapes, dimensions, and compositions. This spectroscopy method is being used to investigate fundamental properties and processes of nanoparticles in (bio)-molecular detection devices, or (bio)-imaging tools with improved single-molecule sensitivity. LSPR spectroscopy can provide thermodynamic and real-time kinetic data for binding processes. LSPR-based tools will be helpful to analyze faster and with higher sensitivity. The application of LSPR spectroscopy is mainly used for biological and chemical sensing by transducing changes in the local refractive index via a wavelength-shift measurement, due to its sensitivity, wavelength tunability, smaller sensing volumes, and lower instrumentation cost. Single-nanoparticle LSPR spectroscopy is an important tool for understanding the relationship between local structure and spectra. In addition, single nanoparticles can provide even higher refractive-index sensitivity than nanoparticle arrays.
4. Properties of AgNPs
Physical and chemical properties of AgNPs—including surface chemistry, size, size distribution, shape, particle morphology, particle composition, coating/capping, agglomeration, dissolution rate, particle reactivity in solution, efficiency of ion release, cell type, and finally type of reducing agents used for synthesis—are crucial factors for determination of cytotoxicity [ 15 , 50 , 167 , 168 , 169 , 170 , 171 , 172 , 173 , 174 , 175 , 176 ]. For example, using biological reducing agents such as culture supernatants of various Bacillus species, AgNPs can be synthesized in various shapes, such as spherical, rod, octagonal, hexagonal, triangle, flower-like, and so on ( Figure 2 ). Previous studies supported the assertion that smaller size particles could cause more toxicity than larger, because they have larger surface area [ 176 ]. Shape is equally important to the determination of toxicity [ 177 ]. For example, in the biomedical field, various types of nanostructures have been used, including nanocubes, nanoplates, nanorods, spherical nanoparticles, flower-like, and so on [ 175 , 178 ]. AgNP toxicity mainly depends on the availability of chemical and or biological coatings on the nanoparticle surface [ 179 ]. AgNP surface charges could determine the toxicity effect in cells. For instance, the positive surface charge of these NPs renders them more suitable, allowing them to stay for a long time in blood stream compared to negatively-charged NPs [ 180 ], which is a major route for the administration of anticancer agents [ 181 , 182 ].

Biological synthesis of various shapes of AgNPs using culture supernatant of various Bacillus species. ( A ) Spherical; ( B ) mixed populations (octagonal, rod, hexagonal, and icosahedral); ( C ) highly branched; ( D ) flower-like in shape.
5. Biological Applications of AgNPs
Due to their unique properties, AgNPs have been used extensively in house-hold utensils, the health care industry, and in food storage, environmental, and biomedical applications. Several reviews and book chapters have been dedicated in various areas of the application of AgNPs. Herein, we are interested in emphasizing the applications of AgNPs in various biological and biomedical applications, such as antibacterial, antifungal, antiviral, anti-inflammatory, anti-cancer, and anti-angiogenic. Herein, we specifically addressed previously-published seminal papers and end with recent updates. A schematic diagram representing various applications of AgNPs is provided in Figure 3 .

Various applications of AgNPs.
5.1. Antibacterial Activity of AgNPs
AgNPs seem to be alternative antibacterial agents to antibiotics and have the ability to overcome the bacterial resistance against antibiotics. Therefore, it is necessary to develop AgNPs as antibacterial agents. Among the several promising nanomaterials, AgNPs seem to be potential antibacterial agents due to their large surface-to-volume ratios and crystallographic surface structure. The seminal paper reported by Sondi and Salopek-Sondi [ 6 ] demonstrated the antimicrobial activity of AgNPs against Escherichia coli , in which E. coli cells treated with AgNPs showed the accumulation of AgNPs in the cell wall and the formation of “pits” in the bacterial cell walls, eventually leading to cell death. In the same E. coli strain, smaller particles with a larger surface-to-volume ratio showed a more efficient antibacterial activity than larger particles [ 183 ]. Furthermore, the antibacterial activity of AgNPs is not only size—but also shape-dependent [ 70 ]. AgNPs were synthesized by four different types of saccharides with an average size of 25 nm, showing high antimicrobial and bactericidal activity against Gram-positive and Gram-negative bacteria, including highly multi-resistant strains such as methicillin-resistant Staphylococcus aureus . As mentioned previously, not only the size is important for determining the efficiency, but also shape, because AgNPs undergo a shape-dependent interaction with the Gram-negative organism E. coli [ 71 ]. Furthermore, a detailed study was carried out to investigate the efficiency of the antimicrobial effects of AgNPs against yeast, E. coli , and Staphylococcus aureus . The results suggest that at low concentrations of AgNPs, the complete inhibition of growth was observed in yeast and E. coli , whereas a mild effect was observed in S. aureus [ 184 ]. Biologically synthesized AgNPs from the culture supernatants of Klebsiella pneumoniae were evaluated; the efficiencies of various antibiotics, such as penicillin G, amoxicillin, erythromycin, clindamycin, and vancomycin against Staphylococcus aureus and E. coli were increased in the presence of Ag-NPs [ 185 ]. When compared to AgNPs, hydrogel–silver nanocomposites showed excellent antibacterial activity against E. coli . One-pot synthesis of chitosan–Ag–nanoparticle composite was found to have higher antimicrobial activity than its components at their respective concentrations, because one-pot synthesis favors the formation of small AgNPs attached to the polymer, which can be dispersed in media of pH ≤ 6.3 [ 186 ]. Biologically produced AgNPs using culture supernatants of Staphylococcus aureus showed significant antimicrobial activity against methicillin-resistant S. aureus , followed by methicillin-resistant Staphylococcus epidermidis and Streptococcus pyogenes , whereas only moderate antimicrobial activity was observed against Salmonella typhi and Klebsiella pneumoniae [ 187 ]. The mechanisms of AgNP-induced cell death was observed in E. coli through the leakage of reducing sugars and proteins. Furthermore, AgNPs are able to destroy the permeability of the bacterial membranes via the generation of many pits and gaps, indicating that AgNPs could damage the structure of the bacterial cell membrane [ 2 ]. Silver nanocrystalline chlorhexidine (AgCHX) complex showed strong antibacterial activity against the tested Gram-positive/negative and methicillin-resistant Staphylococcus aureus (MRSA) strains. Interestingly, the minimal inhibitory concentrations (MICs) of nanocrystalline Ag(III)CHX were much lower than those of the ligand (CHX), AgNO 3 , and the gold standard, silver sulfadiazine [ 188 ].
Biofilms are not only leads to antimicrobial resistance, but are involved in the development of ocular-related infectious diseases, such as microbial keratitis [ 189 ]. Kalishwaralal and co-workers demonstrated the potential anti-biofilm activity against Pseudomonas aeruginosa and Staphylococcus epidermidis . Similarly, guava leaf extract reduced AgNPs (Gr-Ag-NPs) showed significant antibacterial activity and stability against E. coli compared to chemically synthesized AgNPs; the reason for this higher activity could be the adsorption of biomolecules on the surface of the Gr-Ag-NPs [ 190 ]. AgNPs synthesized by Cryphonectria sp. showed antibacterial activity against various human pathogenic bacteria, including S. aureus , E. coli , Salmonella typhi , and Candida albicans . Interestingly, these particular AgNPs exhibited higher antibacterial activity against both S. aureus and E. coli than against S. typhi and C. albicans . Figure 4 shows the effectiveness of dose-dependent antibacterial activity of biologically synthesized AgNPs in E. coli.

Dose-dependent antibacterial activity of biologically synthesized AgNPs in E. coli. CON: control.
Besinis et al. [ 191 ] compared the toxic efficiency of different nanomaterials, such as AgNPs, silver, and titanium dioxide against routine disinfectant chlorhexidine in Streptococcus mutans . Among various nanomaterials, AgNPs had the strongest antibacterial activity of the NPs tested. Agnihotri et al. [ 192 ] demonstrated that the mechanisms of AgNPs on bactericidal action using AgNPs immobilized on an amine-functionalized silica surface. They found that contact killing is the predominant bactericidal mechanism, and surface-immobilized nanoparticles show greater efficacy than colloidal AgNPs, as well as a higher concentration of silver ions in solution. The nanocomposite containing silver/polyrhodanine nanocomposite-decorated silica nanoparticles shows potential and enhanced antibacterial activity against E. coli and S. aureus , which is due to the particular combination of AgNPs and the polyrhodanine [ 155 ]. Interestingly, Khurana et al. [ 193 ] investigated the antimicrobial properties of silver based on its physical and surface properties against S. aureus , B. megaterium , P. vulgaris , and S. sonnei . The enhancement of antibacterial action was observed with particles having a hydrodynamic size of 59 nm compared to 83 nm. Gurunathan et al. [ 68 ] reported that the antibacterial and anti-biofilm activity of antibiotics, AgNPs, or combinations of AgNPs against important pathogenic bacteria Pseudomonas aeruginosa , Shigella flexneri , Staphylococcus aureus , and Streptococcus pneumoniae . The results suggest that, the combination of both antibiotics and AgNPs showed significant antimicrobial and anti-biofilm effects at the lowest concentration of antibiotics and AgNPs compared to AgNPs or antibiotics alone. Nanocomposite spheres composed of AgNPs decorated on the polymer colloids exhibited excellent antibacterial activity [ 194 ]. Recently, nanocomposites containing graphene and AgNPs showed much interest in antibacterial activity. Graphene oxide (GO)-Ag nanocomposite showed enhanced antibacterial activity against E. coli and S. aureus using the conventional plate count method and disk diffusion method [ 195 ]. The GO-Ag nanocomposite exhibited an excellent antibacterial activity against methicillin-resistant S. aureus , Acinetobacter baumannii , Enterococcus faecalis , and Escherichia coli . In addition, GO-Ag nanocomposite is a promising antibacterial agent against common nosocomial bacteria, particularly antibiotic-resistant MRSA [ 196 ]. AgNPs derived from fungal extracts as reducing agents (F-AgNPs) showed enhanced antibacterial activity both in Pseudomonas aeruginosa and Staphylococcus aureus when compared to AgNPs derived from the culture supernatant of bacteria (B-AgNPs) ( Figure 5 ). The minimum inhibitory concentration of F-AgNPs is lesser than B-AgNPs. Nano-silver interacts with peptides and bacteria and serves as nanomedicine in various bacteria, fungi, and virus-mediated diseases [ 197 ].

Differential antibacterial activity of AgNPs synthesized with Calocybe indica extracts (F-AgNPs) and the culture supernatant of Bacillus tequilensis (B-AgNPs) as reducing agents.
5.2. Antifungal Activity of AgNPs
Fungal infections are more frequent in patients who are immunosuppressed, and overcoming fungi-mediated diseases is a tedious process, because currently there is a limited number of available antifungal drugs [ 198 ]. Therefore, there is an inevitable and urgent need to develop antifungal agents, which should be biocompatible, non-toxic, and environmentally friendly. At this juncture, AgNPs play an important role as anti-fungal agents against various diseases caused by fungi. Nano-Ag showed potent anti-fungal activity against clinical isolates and ATCC strains of Trichophyton mentagrophytes and Candida species with concentrations of 1–7 μg/mL. Esteban-Tejeda et al. [ 199 ] developed an inert matrix containing AgNPs with an average size of 20 nm into a soda-lime glass which shows enhanced biocidal activity. Monodisperse Nano-Ag sepiolite fibers showed significant antifungal activity against Issatchenkia orientalis . AgNPs exhibited good antifungal activity against Aspergillus niger and a MIC of 25 μg/mL against Candida albicans [ 200 ]. Biologically-synthesized AgNPs showed enhanced antifungal activity with fluconazole against Phoma glomerata , Phoma herbarum , Fusarium semitectum , Trichoderma sp., and Candida albicans [ 201 ]. AgNPs stabilized by sodium dodecyl sulfate showed enhanced antifungal activity against Candida albicans compared to conventional antifungal agents [ 20 ]. The size-dependent antifungal activities of different AgNPs were performed against mature Candida albicans and Candida glabrata biofilms. Biologically synthesized AgNPs exhibited antifungal activity against several phytopathogenic fungi, including Alternaria alternata , Sclerotinia sclerotiorum , Macrophomina phaseolina , Rhizoctonia solani , Botrytis cinerea , and Curvularia lunata at the concentration of 15 mg [ 202 , 203 ]. Similarly, The AgNPs synthesized by Bacillus species exhibited strong antifungal activity against the plant pathogenic fungus Fusarium oxysporum at the concentration of 8 μg/mL [ 204 ]. Carbon nanoscrolls (CNSs) composed of graphene oxides and AgNPs exhibited enhanced and prolonged antifungal activity against Candida albicans and Candida tropical compared to GO–AgNP nanocomposites containing graphene oxide and AgNPs [ 205 ]. The antifungal efficacy of AgNPs was evaluated in combination with nystatin (NYT) or chlorhexidine (CHX) against Candida albicans and Candida glabrata biofilms. The results from this investigation suggest that AgNPs combined with either nystatin (NYT) or chlorhexidine digluconate (CHG) showed better synergistic anti-biofilm activity; however, this activity depends on the species and drug concentrations [ 206 ].
The biologically synthesized AgNPs exhibited strong antifungal activity against Bipolaris sorokiniana by the inhibition of conidial germination [ 207 ]. Interestingly, AgNPs not only inhibit human and plant pathogenic fungi, but also indoor fungal species such as Penicillium brevicompactum , Aspergillus fumigatus , Cladosporium cladosporoides , Chaetomium globosum , Stachybotrys chartarum , and Mortierella alpine cultured on agar media [ 208 ].
5.3. Antiviral Activity of AgNPs
Viral mediated diseases are common and becoming more prominent in the world; therefore, developing anti-viral agents is essential. The mechanisms of the antiviral activity of AgNPs are an important aspect in antiviral therapy. AgNPs have unique interactions with bacteria and viruses based on certain size ranges and shapes [ 70 , 209 , 210 ]. The antiviral activity nano-Ag incorporated into polysulfone ultrafiltration membranes (nAg-PSf) was evaluated against MS2 bacteriophage, which shows that significant antiviral activity was a result of increased membrane hydrophilicity [ 21 ]. Lara et al. [ 211 ] showed the first mechanistic study demonstrating anti-HIV activity at an early stage of viral replication. Poly vinyl pyrrolidone (PVP)-coated AgNPs prevented the transmission of cell-associated HIV-1 and cell-free HIV-1 isolates [ 211 ]. AgNPs have demonstrated efficient inhibitory activities against human immunodeficiency virus (HIV) and hepatitis B virus (HBV) [ 212 ]. A study was attempted to investigate the antiviral action of the AgNPs; the data showed that both macrophage (M)-tropic and T-lymphocyte (T)-tropic strains of HIV-1 were highly sensitive to the AgNP-coated polyurethane condom (PUC) [ 213 ]. Although several studies have shown that AgNPs could inhibit the viability of viruses, the exact mechanism of antiviral activity is still obscure. However, the studies from Trefry and Wooley found that AgNPs caused a four- to five-log reduction in viral titer at concentrations that were not toxic to cells [ 214 ]. Interestingly, in the presence of AgNPs, virus was capable of adsorbing to cells, and this viral entry is responsible for the antiviral effects of AgNPs. Hemagglutination assay indicated that AgNPs could significantly inhibit the growth of the influenza virus in Madin-Darby canine kidney cells. The study from intranasal AgNP administration in mice significantly enhanced survival, lower lung viral titer levels, minor pathologic lesions in lung tissue, and remarkable survival advantage after infection with the H3N2 influenza virus, suggesting that AgNPs had a significant role in mice survival [ 215 ]. Biologically-synthesized AgNPs inhibited the viability in herpes simplex virus (HSV) types 1 and 2 and human parainfluenza virus type 3, based on size and zeta potential of AgNPs [ 216 ]. The treatment of Vero cells with non-cytotoxic concentrations of AgNPs significantly inhibited the replication of Peste des petits ruminants virus (PPRV). The mechanisms of viral replication are due to the interaction of AgNPs with the virion surface and the virion core [ 217 ]. Tannic acid mediated the synthesis of various sizes of AgNPs capable of reducing HSV-2 infectivity both in vitro and in vivo through direct interaction, blocked virus attachment, penetration, and further spread [ 218 ]. The antiviral property of Ag + alone and a combination of 50 ppb Ag + and 20 ppm CO 3 2− (carbonate ions) was performed on bacteriophage MS2 phage. The results from this study showed that 50 ppb Ag + alone was unable to affect the phage, and the combination of 50 ppb Ag + and 20 ppm CO 3 was found to have an effective antiviral property within a contact time of 15 min [ 219 ]. Treatment with AgNPs for 24 h in Bean Yellow Mosaic Virus (BYMV) decreased virus concentration, percentage of infection, and disease severity [ 220 ].
5.4. Anti-Inflammatory Activity of AgNPs
Inflammation is an early immunological response against foreign particles by tissue, which is supported by the enhanced production of pro-inflammatory cytokines, the activation of the immune system, and the release of prostaglandins and chemotactic substances such as complement factors, interleukin-1 (IL-1), TNF-α, and TGF-β [ 221 , 222 , 223 , 224 ]. In order to overcome inflammatory action, we need to find effective anti-inflammatory agents. Among several anti-inflammatory agents, AgNPs have recently played an important role in anti-inflammatory field. AgNPs have been known to be antimicrobial, but the anti-inflammatory responses of AgNPs are still limited. Bhol and Schechter [ 225 ] reported the anti-inflammatory activity in rat. Rats treated intra-colonically with 4 mg/kg or orally with 40 mg/kg of nanocrystalline silver (NPI 32101) showed significantly reduced colonic inflammation. Mice treated with AgNPs showed rapid healing and improved cosmetic appearance, occurring in a dose-dependent manner. Furthermore, AgNPs showed significant antimicrobial properties, reduction in wound inflammation, and modulation of fibrogenic cytokines [ 226 ]. Continuing the previous study, Wong et al. [ 222 ] investigated to gain further evidence for the anti-inflammatory properties of AgNPs, in which they used both in vivo and in vitro models and found that AgNPs are able to down-regulate the quantities of inflammatory markers, suggesting that AgNPs could suppress inflammatory events in the early phases of wound healing [ 222 ]. A porcine contact dermatitis model showed that treatment with nanosilver significantly increases apoptosis in the inflammatory cells and decreased the levels of pro-inflammatory cytokines [ 227 ]. Biologically-synthesized AgNPs can inhibit the production of cytokines induced by UV-B irradiation in HaCaT cells, and also reduces the edema and cytokine levels in the paw tissues [ 228 ].
5.5. Anti-Angiogenic Activity of AgNPs
Pathological angiogenesis is a symbol of cancer and various ischemic and inflammatory diseases [ 229 ]. There are several research groups interested in discovering novel pro- and anti-angiogenic molecules to overcome angiogenic-related diseases. Although there are several synthetic molecules having anti-angiogenic properties, the discovery of a series of natural pro- and anti-angiogenic factors suggests that this may provide a more physiological approach to treat both classes of angiogenesis-dependent diseases in the near future [ 230 ]. Recently, several studies provided supporting evidence using both in vitro and in vivo models showing that AgNPs have both anti-angiogenic and anti-cancer properties. Herein, we wish to summarize the important contribution in cancer and other angiogenic related diseases.
Kalishwaralal et al. [ 231 ] demonstrated the anti-angiogenic property of biologically synthesized AgNPs using bovine retinal endothelial cells (BRECs) as a model system, in which they found the inhibition of proliferation and migration in BRECs after 24 h of treatment with AgNPs at 500 nM concentration. The mechanisms of inhibition of vascular endothelial growth factor (VEGF) induced angiogenic process by the activation of caspase-3 and DNA fragmentation, and AgNPs inhibited the VEGF-induced PI3K/Akt pathway in BRECs [ 232 ]. Followed by this study, Gurunathan et al. [ 23 ] provided evidence for the anti-angiogenic property of AgNPs by using pigment epithelium derived factor (PEDF) as a bench mark, which is known as a potent anti-angiogenic agent. Using BRECs as an in vitro model system, they found that AgNPs inhibited VEGF-induced angiogenic assays. Furthermore, they demonstrated that AgNPs could block the formation of new blood microvessels by the inactivation of PI3K/Akt. The same group also demonstrated the anticancer property of AgNPs using various cytotoxicity assays in Dalton’s lymphoma ascites (DLA) cells, and a tumor mouse model showed significantly increased survival time in the presence of AgNPs [ 24 ]. AgNPs reduced with diaminopyridinyl (DAP)-derivatized heparin (HP) polysaccharides (DAPHP) inhibited basic fibroblast growth factor (FGF-2)-induced angiogenesis compared to glucose conjugation [ 232 ]. Kim et al. [ 233 ] developed an anti-angiogenic Flt1 peptide conjugated to tetra- N -butyl ammonium modified hyaluronate (HA-TBA), and it was used to encapsulate genistein. Using human umbilical vein endothelial cells (HUVECs) as in vitro model system, they found that genistein/Flt1 peptide–HA micelle inhibited the proliferation of HUVECs, and the same reagents could drastically reduce corneal neovascularization in silver nitrate-cauterized corneas of Sprague Dawley (SD) rats. Ag 2 S quantum dots (QDs) used as nanoprobes to monitor lymphatic drainage and vascular networks. Ag 2 S-based nanoprobes showed long circulation time and high stability. In addition, they were able to track angiogenesis mediated by a tiny tumor (2–3 mm in diameter) in vivo [ 5 ]. Recently, Achillea biebersteinii flowers extract-mediated synthesis of AgNPs containing a concentration of 200 μg/mL showed a 50% reduction in newly-formed vessels [ 234 ]. Figure 6 shows the inhibitory effect of AgNPs on VEGF induced angiogenic activity in bovine retinal endothelial cells (BRECs) and human breast cancer cells MDA-MB 231.

Effect of AgNPs on vascular endothelial growth factor (VEGF)-induced proliferation of ( A ) bovine retinal endothelial cells (BRECs); and ( B ) human breast cancer cells MDA-MB 231. Cells were treated with VEGF with or without AgNPs for 24 h. Cell proliferation was determined by trypan blue exclusion assay.
5.6. Anticancer Activity of AgNPs
In our lifetime, 1 in 3 people has the possibility to develop cancer [ 235 ]. Although many chemotherapeutic agents are currently being used on different types of cancers, the side effects are enormous, and particularly, administrations of chemotherapeutic agents by intravenous infusion are a tedious process [ 235 ]. Therefore, it is indispensable to develop technologies to avoid systemic side effects. At this juncture, many researchers are interested in developing nanomaterials as an alternative tool to create formulations that can target tumor cells specifically. Several research laboratories have used various cell lines to address the possibility of finding a new molecule to battle cancer. Here we summarized the work from various laboratories reporting anticancer activity using both in vitro and in vivo model systems. Gopinath et al. [ 236 ] investigated the molecular mechanism of AgNPs and found that programmed cell death was concentration-dependent under conditions. Further, they observed a synergistic effect on apoptosis using uracil phosphoribosyltransferase (UPRT)-expressing cells and non-UPRT-expressing cells in the presence of fluorouracil (5-FU). In these experimental conditions, they observed that AgNPs not only induce apoptosis but also sensitize cancer cells. The anticancer property of starch-coated AgNPs was studied in normal human lung fibroblast cells (IMR-90) and human glioblastoma cells (U251). AgNPs induced alterations in cell morphology, decreased cell viability and metabolic activity, and increased oxidative stress leading to mitochondrial damage and increased production of reactive oxygen species (ROS), ending with DNA damage. Among these two cell types, U251 cells showed more sensitivity than IMR-90 [ 237 ]. The same group also demonstrated that the cellular uptake of AgNPs occurred mainly through endocytosis. AgNP-treated cells exhibited various abnormalities, including upregulation of metallothionein, downregulation of major actin binding protein, filamin, and mitotic arrest [ 237 ]. The morphology analysis of cancer cells suggests that biologically synthesized AgNPs could induce cell death very significantly. Jun et al. [ 238 ] elegantly prepared multifunctional silver-embedded magnetic nanoparticles, in which the first type consist of silver-embedded magnetic NPs with a magnetic core of average size 18 nm and another type consist of a thick silica shell with silver having an average size of 16 nm; the resulting silica-encapsulated magnetic NPs (M-SERS dots) produce strong surface-enhanced Raman scattering (SERS) signals and have magnetic properties, and these two significant properties were used for targeting breast-cancer cells (SKBR3) and floating leukemia cells (SP2/O).
The antineoplastic activities of protein-conjugated silver sulfide nano-crystals are size dependent in human hepatocellular carcinoma Bel-7402 and C6 glioma cells [ 239 ]. Instead of giving direct treatment of AgNPs into the cells, some researchers developed chitosan as a carrier molecule for the delivery of silver to the cancer cells. For example, Sanpui et al. [ 240 ] demonstrated that chitosan-based nanocarrier (NC) delivery of AgNPs induces apoptosis at very low concentrations. They then examined cytotoxic efficiency using a battery of biochemical assays. They found an increased level of intracellular ROSin HT 29 cells. Lower concentrations of nanocarrier with AgNPs showed better inhibitory results than AgNPs alone. Boca et al. [ 241 ] reported that chitosan-coated silver nanotriangles (Chit-AgNTs) show an increased cell mortality rate. In addition, human embryonic cells (HEK) were able to take up Chit-AgNTs efficiently, and the cytotoxic effect of various sizes of AgNPs was significant in acute myeloid leukemia (AML) cells [ 242 ]. Recently, the anticancer property of bacterial (B-AgNPs) and fungal extract-produced AgNPs (F-AgNPs) was demonstrated in human breast cancer MDA-MB-231 cells. Both biologically produced AgNPs exhibited significant cytotoxicity [ 62 , 243 ]. Among these two AgNPs, fungal extract-derived AgNPs had a stronger effect than B-AgNPs, which is due to the type of reducing agents used for the synthesis of AgNPs. Similarly, AgNPs derived from Escherichia fergusoni showed dose-dependent cytotoxicity against MCF-7 cells [ 62 ]. Plant extract-mediated synthesis of AgNPs showed more pronounced toxic effect in human lung carcinoma cells (A549) than non-cancer cells like human lung cells, indicating that AgNPs could target cell-specific toxicity, which could be the lower level of pH in the cancer cells [ 63 ]. Targeted delivery is an essential process for the treatment of cancer. To address this issue, Locatelli et al. [ 244 ] developed multifunctional nanocomposites containing polymeric nanoparticles (PNPs) containing alisertib (Ali) and AgNPs. PNPs conjugated with a chlorotoxin (Ali@PNPs–Cltx) showed a nonlinear dose–effect relationship, whereas the toxicity of Ag/Ali@PNPs–Cltx remained stable. Biologically synthesized AgNPs showed significant toxicity in MCF7 and T47D cancer cells by higher endocytic activity than MCF10-A normal breast cell line [ 245 ]. Banti and Hadjikakou explained the detailed account of anti-proliferative and anti-tumor activity of silver(I) compounds [ 246 ]. Biologically synthesized AgNPs capable of altering cell morphology of cancer cells ( Figure 7 ), which is an early indicator for apoptosis. Apoptosis can be determined by structural alterations in cells by transmitted light microscopy.

Anticancer activity of biologically synthesized AgNPs using Bacillus species in human ovarian cancer and human breast cancer cells.
5.7. Diagnostic, Biosensor, and Gene Therapy Applications of AgNPs
The advancement in medical technologies is increasing. There is much interest in using nanoparticles to improve or replace today’s therapies. Nanoparticles have advantages over today’s therapies, because they can be engineered to have certain properties or to behave in a certain way. Recent developments in nanotechnology are the use of nanoparticles in the development of new and effective medical diagnostics and treatments. The ability of AgNPs in cellular imaging in vivo could be very useful for studying inflammation, tumors, immune response, and the effects of stem cell therapy, in which contrast agents were conjugated or encapsulated to nanoparticles through surface modification and bioconjugation of the nanoparticles. Silver plays an important role in imaging systems due its stronger and sharper plasmon resonance. AgNPs, due to their smaller size, are mainly used in diagnostics, therapy, as well as combined therapy and diagnostic approaches by increasing the acoustic reflectivity, ultimately leading to an increase in brightness and the creation of a clearer image [ 247 , 248 ]. Nanosilver has been intensively used in several applications, including diagnosis and treatment of cancer and as drug carriers [ 249 , 250 , 251 ]. Nanosilver was used in combination with vanadium oxide in battery cell components to improve the battery performance in next-generation active implantable medical devices [ 250 ]. Recently, silver has been used to develop silver-based biosensors for the clinical detection of serum p53 in head and neck squamous cell carcinoma [ 252 ]. In addition, it has been explored for the location of cancer cells, and can absorb light and selectively destroy targeted cancer cells through photothermal therapy [ 253 ].
6. Mechanism of the Anti-Cancer Activity of AgNPs
The next interesting aspect of silver is to find out the mechanism of AgNP-induced apoptosis in cancer cells. In this context, AshaRani et al. [ 254 ] investigated the cellular and molecular mechanisms of nanoparticle-induced effects using normal human lung cells IMR-90 and human brain cancer cells U251. They found that AgNPs were capable of adsorbing cytosolic proteins on their surface that may influence the function of intracellular factors, and that they can regulate gene expression and pro-inflammatory cytokines. Foldbjerg et al. [ 255 ] addressed the interesting aspect of cellular transcriptome analysis upon exposure of human lung epithelial cell line A549 using microarray analysis. The results from this study exhibited that AgNPs could alter the regulation of more than 1000 genes. Among several genes, metallothionein, heat shock protein, and histone families were significant [ 255 ]. Recently, autophagy-induced cell death has been another identified mechanism for the anti-cancer activity of AgNPs. Autophagy induced by nanoparticles is a critical cellular degradation process, and elevated autophagy could promote cell death [ 256 ]. Our recent findings show that AgNPs are capable of inducing autophagy through the accumulation of autophagolysosmes in human ovarian cancer cells ( Figure 8 ). Therefore, autophagy can have a dual function; at lower levels, it can enhance the cell survival, and at elevated levels, it can cause cell death. Therefore, the use of autophagy inhibitors or autophagy protein-5 (ATG5)—small interfering RNAs (siRNA) enhanced AgNPs induced cell death in cancer cells.

Biologically synthesized AgNPs using Bacillus species induce accumulation of autophagolysosomes in human ovarian cancer cells.
The antitumor activity of AgNPs was significantly enhanced in B16 mouse melanoma cells by the inhibition of autophagy using wortmanin [ 256 ]. One of the most important mechanisms of the toxicity of AgNPs is that excessive levels of intracellular ion concentration increases the production of ROS, which is produced by cellular oxygen metabolism [ 15 , 68 ]. On other hand, uncontrolled ROS production can lead to serious cellular injuries [ 15 ], such as DNA damage and mitochondria-involved apoptotic cell death [ 1 , 257 , 258 , 259 ]. Recently, De Matteis et al. [ 260 ] proposed that endocytosed AgNPs are degraded in the lysosomes, and the release of Ag + ions in the cytosol induces cell damages. Hatipoglu et al. [ 261 ] revealed that the cytotoxicity and genotoxicity of AgNPs depends on reaction times for the preparation of AgNPs. The conclusion drawn from this study suggests that AgNP seeds were the major source of toxicity. Gurunathan et al. [ 1 ] reported that cytotoxicity of AgNPs in human breast cancer cells MDA-MB-231 via the activation of p53, p-Erk1/2, and caspase-3 signaling, and the downregulation of Bcl-2. Importantly, AgNP-mediated apoptosis was a p53-dependent pathway. On the other hand, Zuberek et al. [ 262 ] demonstrated that AgNPs not only induced oxidative stress, but also indicated the influence of energy supply from glucose availability in the media. They showed evidence by growing HepG2 cells in two different media with high (25 mM) or low (5.5 mM) glucose content in the presence of 20 nm AgNPs. In this assay, they observed that AgNPs induced the dose-dependent generation of H 2 O 2 . The study suggests that lower levels of glucose are responsible for defense mechanisms. All of these studies suggest that AgNPs can induce cell death through various processes, including ROS generation, enhanced leakage of lactate dehydrogenase, upregulation of apoptosis and autophagy genes, endoplasmic reticulum stress, mitochondrial dysfunction, activation of caspases, and DNA damage.
7. Therapeutic Approach for Cancer Treatment Using AgNPs
The application of AgNPs in cancer is divided into diagnostic and therapeutic purposes. Several laboratories have addressed the enhancement of the therapeutic usage of AgNPs as nanocarriers for targeted delivery, chemotherapeutic agents, and as enhancers for radiation and photodynamic therapy. Here we summarized the possible therapeutic approaches for cancer using AgNPs in cancer cell lines or animal models. For instance, Lim et al. [ 263 ] synthesized plasmonic magnetic nanoparticles to enhance MRI contrast consisting of multiple components of various nanoparticles in a single platform containing silver monolayer- gold-coated magnetic nanoparticles. These coated materials showed highly efficient killing of SKBr3 cells within 3 min of near-infrared laser at the relatively low exposure of 12.7 W/cm at 808 nm. To address the efficiency of photothermal therapy, Huang et al. [ 264 ] designed an aptamer-based nanostructure which combines the high absorption efficiency of Au–Ag nanorods showing excellent hyperthermia efficiency and selectivity. The combination of AgNPs with ligands strongly influences the toxicity and cellular uptake into the cells.
Recently, photo-based nanomedicine has gained much importance for cancer treatment among several approaches [ 265 ]. Khlebtsov et al. [ 266 ] developed multifunctional NPs which significantly induced cell death in HeLa cervical cancer cells. Wang et al. [ 267 ] developed folic acid (FA)-coated AgNPs with an average size of 23 ± 2 nm showing excellent receptor-mediated cellular uptake; with this compound (FA-AgNPs), they conjugated the chemotherapeutic drug doxorubicin (DOX) by electrostatic bonding. DOX was released efficiently, and cell death was observed after 8 h. They concluded that AgNPs can be used as nanocarriers for desired drugs for cancer treatment. To increase intracellular uptake and cytotoxicity in lymphoma cells, Fang et al. [ 268 ] designed self-assembled polymer–doxorubicin conjugates such as NP-Im/DOX, NP-Ag/DOX, and NP-Dm/DOX (Nanoparticles (NP), guanidinium group (Ag), an imidazole group (Im), and a tertiary amine group (Dm), doxorubicin (Dox)) using three different cationic side chains with an average of 80 nm for the efficient delivery of nanocarrier. Locatelli et al. [ 269 ] developed a nanocarrier by using a simple method, in which lipophilic AgNPs entrapped into PEG-based polymeric nanoparticles containing chlorotoxin. The interesting aspect using this nanocarrier showed enhanced cellular uptake and cytotoxic effect.
Recently, nanomaterials have been used for diagnosis, treatment, and prevention of cancer using photo-based therapeutic approaches [ 270 ]. The nanostructures are more capable of destroying the cancer cells than non-cancer cells at low irradiation power density [ 271 ]. In this context, Wu et al. [ 271 ] developed sensitive and specific detection aptamer-based Ag–Au shell–core nanostructure-photothermal therapy in which the nanostructures were able to target the cells with high affinity and specificity. The intra-tumoral administration of AgNPs in combination with a single dose of ionizing radiation enhanced therapeutic efficiency in C6 glioma-bearing rats [ 272 ]. Nanoparticles consisting of a silver nanoshell with a carbon core composite were significantly cytotoxic to cells in the presence of phototherapy and radiotherapy [ 273 ]. Combination of AgNPs–chitosan–para-aminothiophenol (pATP)–folic acid showed significant stability and targeted uptake in NIH:OVCAR-3 human ovarian cancer cell line. The efficient therapeutic approach was achieved by targeted cancer cell treatment with these composites [ 274 ]. Recently, Mukherjee et al. [ 275 ] used AgNPs as cancer theranostic agents; they prepared AgNPs from Olax scandens leaf extract and prepared AgNPs showing anticancer activity against various types of cancer cells, including A549, B16, and MCF7. Furthermore, they observed a bright red fluorescence signal from AgNPs, which can be exploited for localized drug delivery into the cancer cells. Considering the literature, AgNPs are not only used as drug delivery devices but they also serve as drugs; therefore, they are used for therapeutics [ 276 ]. AgNPs are well known antibacterial agents, and they also enhance the tumor-killing effects of anticancer drugs [ 276 ]. The combination of chemotherapeutic agents with nanoparticles is a developing effective approach for the eradication of cancer, in which they are using lower doses of drugs to reduce cytotoxic effects and increase the efficacy [ 277 ]. For example, the combination of Platinol (cisplatin) and Navelbine (vinorelbine) showed better efficiency in non-small cell lung cancer [ 278 ]. Combination of CPX-351 in a liposomal NP with cytarabine and daunorubicin showed better efficacy in the treatment of acute myeloid leukemia [ 279 ]. Similarly, the combination of salinomycin (Sal) with AgNPs derived from Typha angustifolia plant extracts showed a synergistic cytotoxic effect in human ovarian cancer cells ( Figure 9 ).

Morphological changes of the human ovarian cancer cell line A2780 after treatment with Salinimycin (Sal), AgNPs, and Sal plus AgNPs. A2780 cells were treated with Sal (3 μM), AgNPs (3μg/mL), and Sal plus AgNPs (3 μM plus 3 μg/mL) for 24 h, and the morphological changes of cells were observed under an inverted microscope (200×). The combination of Sal and AgNP induced significant morphological changes.
Altogether, published literatures suggest that the AgNPs is a suitable promising agent to inhibit the growth of cancer cells via various mechanistic approaches. The hypothetical mechanism is shown in Figure 10 .

The possible mechanisms of AgNP-induced cytotoxicity in cancer cell lines. Endoplasmic reticulum stress(ER), lactate dehydrogenase (LDH), reactive oxygen species (ROS).
8. Challenges for Cancer Therapy Using AgNPs
Nanomedicine is as one of the fast developing and promising strategies to combat cancer using metallic nanoparticles. Current treatment for cancer, such as chemo- and radiation therapy, has limitations due to unexpected drug-associated side effects, lack of specificity of low drug concentrations at the tumor target site, and the development of chemoresistance [ 280 , 281 ]. Nanoparticle-mediated therapy is the best, most suitable, and alternative therapeutic strategy in cancer therapy. Nanoparticles (NPs) have the ability to target through passive or active targeting of particular diseased cells or tumor tissues by the encapsulation of therapeutic agents with nanoparticles, and they have been used as drug delivery systems [ 282 ]. Although many nanoparticle-mediated strategies have been developed, heterogeneity of the tumor and its stroma is a significant challenge for nanotechnologists and clinicians to come up with specific formulations to precisely target specific cancer cells. To achieve higher specificity, reduction in toxicity, biocompatibility, safety, better efficacy, and to overcome the limitations of conventional chemotherapy, using new nanoparticles in single platform-based strategies is another challenge in cancer therapy. However, there is a need to address the challenges and limitations of using nanoparticles for cancer therapy; these include physiological barriers, limited carrying capacity, enhanced permeability and retention effect (EPR), variability of nanoparticles, and regulatory and manufacturing issues [ 282 ].
9. Conclusions and Future Perspectives
This review comprehensively addressed synthesis, characterization, and bio-applications of silver nanoparticles, with special emphasis on anticancer activity and its mechanisms and also therapeutic approaches for cancer using AgNPs. Recently, both academic and industrial research has explored the possibility of using AgNPs as a next-generation anticancer therapeutic agent, due to the conventional side effects of chemo- and radiation therapy. Although AgNPs play an important role in clinical research, several factors need to be considered, including the source of raw materials, the method of production, stability, bio-distribution, controlled release, accumulation, cell-specific targeting, and finally toxicological issues to human beings. The development of AgNPs as anti-angiogenic molecules is one of the most interesting approaches for cancer treatment and other angiogenesis-related diseases; it can overcome poor delivery and the problem of drug resistance. Further, it could provide a new avenue for other angiogenic diseases, such as atherosclerosis, rheumatoid arthritis, diabetic retinopathy, psoriasis, endometriosis, and adiposity.
In addition, the potential use of AgNPs for cancer diagnosis and treatment is immense; to address this issue, a variety of modalities have been developed. Although various methods are available, the synergistic effects of AgNPs and antibiotics on antibacterial agents or multiple therapeutic agents on anti-cancer activity/tumor reduction are still obscure. Therefore, more studies are required to explain the synergistic effect of the two different cytotoxic agents at a single time point. These kinds of studies could provide understanding, mechanisms, and efficiency of the synergistic effect of two different agents or multiple agents; thus, they would help to develop a novel system bearing multiple components with synergistic effects for the treatment of various types of cancer. Although AgNPs have been focused on therapeutic purposes, further research is inevitable in animal models to confirm the mechanisms and to gain a comprehensive picture of biocompatibility vs. toxicity of AgNPs. Finally, if we succeed in all these studies, it would help the researchers of the nanoscience and nanotechnology community to develop safer, biocompatible, efficient cancer or anti-angiogenic agents containing AgNPs. Eventually, to ensure the biosafety of the use of AgNPs in humans, studies dealing with biocompatibility of AgNPs and their interaction with cells and tissues are inevitable. Finally, the great concern is that the developing nanotechnology-based therapy should be better than available technologies, and it should overcome the limitations of existing treatment techniques. Finally, it has to provide a safe, reliable, and viable treatment of diseases with high accuracy in a patient-friendly manner.
Acknowledgments
This paper was supported by the KU-Research Professor Program of Konkuk University. This work is also supported by the Science and Technology Research Program from the Department of Education of Hubei Province in China (D20151701). Although we are the authors of this review, we would never have been able to complete it without the great many people who have contributed to the field of nanoparticles research particularly silver nanoparticles. We owe our gratitude to all those researchers who have made this review possible. We wish to thank all the investigators who have contributed to the field of synthesis, characterization, biomedical application and therapeutic approaches of silver nanoparticles. We have cited as many references as permitted, and apologize to the authors of those publications that we have not cited due to limitation of references. We apologize to other authors who have worked on the several aspects of AgNPs, but whom we have unintentionally overlooked.
Author Contributions
Sangiliyandi Gurunathan came up with the idea and participated in writing of the manuscript. Xi-Feng Zhang performed all literature surveys. Wei shen and Zhi-Guo Liu analyzed the interpretation of literature. All authors read and approved the final manuscript.
Conflicts of Interest
The authors declare no conflict of interest.
Advertisement
Potential and risks of nanotechnology applications in COVID-19-related strategies for pandemic control
- Open access
- Published: 09 November 2023
- Volume 25 , article number 229 , ( 2023 )
Cite this article
You have full access to this open access article
- Fatemeh Araste 1 ,
- Astrid Diana Bakker ORCID: orcid.org/0000-0002-0280-9124 2 &
- Behrouz Zandieh-Doulabi ORCID: orcid.org/0000-0002-0290-3241 2
1458 Accesses
2 Altmetric
Explore all metrics
The ongoing battle against viral infections highlighted so recently by the COVID-19 pandemic demonstrates the need to develop new approaches using nanotechnology in antiviral strategies. Nanoparticles have emerged as promising tools in the fight against viral outbreaks, offering various options for application such as biosensors, vaccine nanoparticles, disinfectants, and functionalized nanoparticles. In this comprehensive review, we evaluate the role of nanoparticles in pandemic control, exploring their potential applications, benefits, and associated risks. We first discuss the importance of nanotechnology in viral outbreak management, particularly in vaccine development. Although lipid nanoparticles play a crucial role in mRNA vaccines, there are concerns about their potential side effects. Although functionalization of protective face masks using metallic nanoparticles has emerged as a sustainable alternative to disposable masks, reducing waste production and enhancing virus filtration, improper disposal of such masks leads to environmental contamination and potential ecological harm. Second, we address the potential adverse effects associated with nanoparticle-based vaccines containing polyethylene glycol and other vaccine components, which trigger autoimmune diseases and alter menstrual cycles. To manage outbreaks effectively, we must minimize such potential risks and environmental impacts. Thus, when developing effective strategies for future pandemic control, it is crucial to understand the advantages and challenges associated with nanoparticle usage.
Similar content being viewed by others
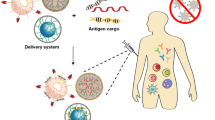
Advances in vaccine delivery systems against viral infectious diseases
Dongyoon Kim, Yina Wu, … Yu-Kyoung Oh
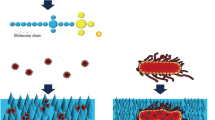
A short review on nanotechnology interventions against COVID-19
Abhimanyu Tharayil, R. Rajakumari, … Nandakumar Kalarikkal
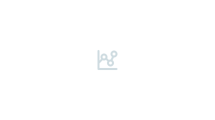
Nanotheranostics and its role in diagnosis, treatment and prevention of COVID-19
Lipsa Leena Panigrahi, Banishree Sahoo & Manoranjan Arakha
Avoid common mistakes on your manuscript.
Introduction
Throughout the twentieth century, viral infections significantly impacted global health, causing millions of deaths worldwide. To combat these diseases, nanotechnology has emerged as a promising approach in the development of antiviral agents such as biosensors, nanoprobes, virus-like particles (VLPs), and functionalized nanoparticles [ 1 , 2 , 3 ]. The COVID-19 pandemic highlighted the importance of nanotechnology in the battle against viral infections, particularly in the development of vaccines. In turn, ongoing discoveries of new virus variants highlight the importance of being prepared to combat potential future pandemics. Recently, for example, three new COVID-related virus variants were discovered in bats in Laos [ 4 ].
In this review, we evaluate the role of nanoparticles in pandemic control and discuss their potential applications, and also the alarms and risks associated with their use. We also draw on the insights learned from the COVID-19 outbreak regarding the use of nanoparticles in managing viral outbreaks. For instance, although antiviral face masks containing metal nanoparticles were proposed as a more sustainable alternative to disposable masks, since they reduce the amount of non-biodegradable waste material [ 5 ], their improper disposal has contributed to the release of metal nanoparticles into the environment. One recent example was their release into Colombian and Southern Brazilian waters [ 6 , 7 ]. Improper disposal may also cause ecological harm [ 8 ]. A comprehensive understanding of the advantages and risks associated with nanoparticle utilization is therefore essential to their responsible and effective use.
Another significant application of nanoparticles in the fight against COVID-19 is the use of lipid nanoparticle platforms for mRNA vaccines. Although the widespread use of lipid nanoparticles containing mRNA vaccines has contributed significantly to pandemic control [ 9 ], the long-term safety and activity of the vaccine-containing nanoparticles are unclear. Neither have the logistics associated with transport — such as special equipment for storage during transport to remote areas, and the costs this involves — been addressed so far.
Below, we explore the various potentials of nanoparticles in viral outbreak management. More specifically, we discuss the use of functionalized face masks for preventing viral infections, the significance of nanoparticle-based drug delivery systems for antiviral therapeutics, and the role of nanoparticles in diagnostic platforms for rapid and accurate viral detection. We also address the concerns and risks associated with the use of nanoparticles, including their potential ecological impacts and other long-term safety considerations. We highlight the critical role of nanoparticles in tackling viral outbreaks, showcasing their potential applications and stressing the importance of their responsible and ethical use. Through proper consideration of the benefits and risks intricately linked with nanoparticle usage, we hope to ensure the development of effective strategies for future pandemic control.
Nanotechnology applications in SARS-CoV-2 transmission prevention
Functionalization of protective face masks using nanoparticles.
The improper disposal of non-reusable face masks has become an environmental concern, as these masks often contain non-biodegradable materials and pathogenic particles in their filter layer. Over time, the mask weathering caused by factors such as mechanical stress, UV-light, or quartz particles causes the release of microplastics from the masks into the environment [ 10 ].
Surgical masks usually consist of three layers, with a central filter layer. To reduce waste production, this filter layer can be functionalized with metallic nanoparticles composed of silver, zinc, or copper, which interfere with viral reproductive cycles, thereby helping to improve virus filtration over that of ordinary masks [ 11 , 12 , 13 , 14 , 15 ]. Metallic nanoparticles can be used to functionalize face masks through the addition of substances such as photo-sensitizing nanoparticles, which produce reactive oxygen species (ROS) upon exposure to specific wavelengths of light, thereby effectively destroying pathogenic membranes, proteins, and nucleic acids after each mask use [ 16 ]. Plasma-based nanoparticles with photo-thermal efficiency, such as graphene, silver, and gold nanoparticles, are able to self-disinfect when exposed to light, and to absorb any moisture present in the mask [ 12 , 17 ]. Graphene-derivatives add other properties to functionalized face masks, such as resistance to smog, mechanical and abrasion stress, and UV light [ 18 ]. Positively charged polymer nanoparticles have strong virucidal properties that reform and fluidize the lipid content of viral membranes, particularly in lipid-raft areas [ 19 ]. Similarly, biodegradable polysaccharide-based materials successfully combat COVID-19 in facial mask layers, achieving complete decomposition in soil within 4 weeks [ 20 , 21 ].
Various nanotechnology applications in SARS-CoV-2 transmission prevention
As well as functionalizing protective face masks, metallic nanoparticles can be applied in mouthwash and nose rinses, offering new opportunities for combating viral infections. As silver nanoparticles (AgNPs) inhibited SARS-CoV-2 in pre-clinical studies [ 22 , 23 ], they may have potential for wider application. Whereas chemical disinfectants need high concentrations of the active substance, metallic nanoparticles can be used in low concentrations, producing less harmful byproducts and being more effective than standard disinfectants [ 24 ]. Another application of nanotechnology involves spraying nano-sized electrostatic atomized water particles (NEAWPs) onto an electrode on which water molecules have first condensed. This significantly reduces the environmental virus count [ 25 ]. Such use of nanoparticles is particularly important because the excessive use of traditional disinfectants during the pandemic increased levels of quaternary ammonium compounds in water and soil, thereby posing an environmental threat [ 26 ].
Nanoparticles as antiviral therapeutic agents
The main antiviral agents against SARS-CoV-2 infection, i.e., remdesivir, zanamivir, oseltamivir, and abacavir, are specific for HIV and/or influenza, but not for SARS-CoV-2 infections [ 27 , 28 ]. The use of nanoparticles in COVID-19 treatment strategies is promising since the nanoparticles do combat SARS-CoV-2. For example, iron oxide nanoparticles interfere with the S1 subunit of the RBD domain [ 29 ], and amyloid-like proteins from LCB1 and LCB3 sequences of the S protein self-assemble into multivalent spherical nanoparticles, competitively blocking viral interaction with the angiotensin-converting enzyme 2 (ACE2) receptor [ 30 ]. Similarly, linear polyglycerol sulfate and its fullerene-conjugated derivative can block virus entry into host cells [ 31 ]. Biological nanovesicles from human lung spheroid cells that present ACE2, as cell-mimicking nanodecoys, are promising, since they absorb viruses and prevent their attachment to the host cells [ 32 ].
Controlled-release systems of antivirals using nanoparticles
Nanoparticles provide a controlled-release system of antivirals to reduce side effects, increase bioavailability, improve circulation time, or ameliorate delivery of the antivirals [ 33 ]. For example, polymeric nanoparticles made of poly-ε-caprolactone (PCL) or poly-lactic glycolic acid-conjugated-poly-ethylene glycol (PLGA-PEG) decorated with ACE2 ligands successfully encapsulate remdesivir, playing dual antiviral roles through competitive interference with SARS-CoV-2 in ACE2 binding, and through targeted drug delivery to lung cells [ 34 ]. The anti-COVID efficacy of the drug is enhanced by PLGA-lipid hybrid nanoparticles encapsulating fluoxetine hydrochloride [ 35 ]. Table 1 provides information on selected types of nanoparticle that are used against different coronaviruses.
Environmental challenges and urgent action
Adverse effects associated with nanoparticles in personal protective equipment.
Despite some positive aspects of metal nanoparticle usage in personal protective equipment (PPEs) such as masks, potentially adverse effects of metal nanoparticles on ecosystems and their fate in the mask-washing process have not been thoroughly investigated. Their entry into the ecosystem may have unintended consequences.
Due to their high surface-to-volume ratio and reactive surface, nanoparticles are prone to interfere with biological processes. While their environmental impact, particularly that of nanoparticles originating from PPEs, has raised concerns about possible adverse effects on the ecosystem, these effects are not entirely negative. As well as aiding the removal of heavy metals and organic pollutants in wastewater treatment, metal nanoparticles can degrade microplastics and nanoplastics, and produce H 2 O and CO 2 as end-products of degradation [ 8 , 36 , 37 ]. Nonetheless, the prolonged and continuous use of nanoparticles in PPEs leads to the accumulation of toxic levels of degradation products, posing risks to various organisms, and to the entire ecosystem [ 38 ]. In aquatic conditions, metal nanoparticles derived from PPEs can interact with other pollutants. The detrimental effects in organisms range from inflammation to cellular damage that further exacerbate any impact on the environment [ 8 ]. A further problem is the improper disposal of PPEs in landfills, dumpsites, marine environments, or public spaces. This can cause animals to mistakenly recognize such PPE waste as food, resulting in their inadvertent and potentially harmful ingestion.
All in all, urgent action is required to address a range of significant environmental challenges. To promote proper disposal practices and prevent the dissemination of nanoparticles into the environment, specific recycling guidelines tailored to nanotechnology products should be established and enforced [ 19 ]. Before the SARS-CoV-2 pandemic, nanoparticles entered the environment mainly through household usage, industrial waste, or laboratory penetration. However, during the initial stages of the pandemic, when it was believed that the virus could be transmitted through surfaces, the use of antiseptic and disinfectant agents skyrocketed, increasing the release of nanoparticles into the environment. Now, in the post-SARS-CoV-2 period, the main source of nanoparticle pollution is the widespread uncontrolled abandonment of personal protective equipment.
Adverse effects of nanoparticles on plants and microorganisms
During the SARS-CoV-2 pandemic, the worldwide demand for masks reached over 4 billion daily, all while recycling programs for masks were inadequately planned [ 39 ]. Several countries used reusable masks containing carbon nanotubes, and/or silver (Ag), silicon dioxide (SiO 2 ), zinc oxide (ZnO), or nanoparticles titanium dioxide (TiO 2 ). Disposal of nanosilver from these masks was found to pose ecological hazards, inhibiting plant growth and photosynthesis [ 40 ]. Engineered nanoparticles commonly penetrate the roots of plants, resulting in phytotoxicity [ 41 ]. As nanoparticles generate reactive ions interacting with nutrients and inorganic compounds in plants, they cause chlorosis and wilting [ 42 , 43 ]. Small-sized nanoparticles such as TiO 2 pass through protective layers like the cuticle, cell wall, and cell membrane [ 44 ], impairing the growth of seedlings crops, the uptake of minerals, and chlorophyll synthesis [ 45 ]. ZnO nanoparticles reduce chlorophyll production in bulb onions, as well as crop growth and development [ 46 , 47 , 48 ]. Ag nanoparticles increase the activity of antioxidant enzymes, reduce chlorophyll content, and impair photosynthesis in tomatoes [ 49 , 50 ].
Similarly adverse effects of nanoparticles are observed not only in plants, but also in bacteria and aquatic animals [ 51 ]. For example, ZnO-based nanoparticles induce genetic mutations in Caenorhabditis elegans , resulting in offspring toxicity [ 52 , 53 ]. In sea water, TiO 2 nanoparticles released from sunscreens cause severe damage to gill filaments, hampering aquatic animal reproduction [ 54 , 55 ]. Overall, it is therefore clear that the adverse effects of nanoparticles eventually disrupt the food chain for higher organisms.
Adverse effects of nanoparticles on higher organisms
The generation of reactive oxygen species is a biological process. Their excessive generation causes oxidative stress, leading to inflammation, diabetes, cancer, and other degenerative diseases [ 56 , 57 ]. Excessive reactive oxygen species causes free-radical production, lipid peroxidation, genotoxicity, and apoptosis [ 58 ]. Nanoparticles accumulate in various organs and have overall systemic effects [ 59 ]. Some inorganic nanoparticles such as TiO 2 , SiO 2 , ZnO, and Fe 2 O 3 dissolve in the acidic environment of the stomach [ 60 ]. Through absorption into the skin, lungs, and liver [ 61 ], they also impair human health.
Toxicity caused by silver nanoparticles (AgNPs) in vitro depends on the surface coating and the concentration of AGNPs it contains. In vivo, AgNPs enter the bloodstream and accumulate in organs, where they cause cytotoxicity [ 62 ]. The generation of Ag + ions from AgNPs and oxidative stress both lead to apoptosis via translocation of mitochondrial cytochrome C into the cytosol [ 63 ], or to necrosis by reducing sulfhydryl groups [ 64 , 65 ]. Table 2 provides an overview of anti-COVID-19-related cytotoxic effects caused by AgNPs and other types of nanoparticles in vitro and in vivo, and the compendial and noncompendial tests used.
Direct contact with titanium dioxide nanoparticles (TiO 2 NPs) affects skin cells in various ways, for example, by impairing their viability, proliferation, and differentiation [ 66 ]. TiO 2 NPs penetrate into the deep layers of the skin are known to be released in sweat [ 67 , 68 , 69 ]. Inhalation of TiO 2 NPs poses a significant health risk: due to the lower protection provided by the olfactory bulb than by the blood–brain barrier, nano-sized materials can penetrate the brain faster through the olfactory nerve than through systemic injection [ 70 , 71 ].
Being in direct contact with the skin and the air we breathe, protective masks containing Ag and TiO 2 nanoparticles are potentially harmful. The presence of these nanoparticles has been shown to significantly inhibit the growth rate of human osteoblasts, indicating that the adverse effects of the masks are not limited to the skin, inhalation, or brain [ 72 ].
The leaching of Ag + or Cu 2+ ions from metal-impregnated masks has also been linked to potential health risks for humans [ 73 ]. Exposure to these nanoparticles through ingestion, inhalation, or dermal penetration can cause toxicity [ 74 ]. Ingestion is followed by exposure to the complex and harsh condition of the gastrointestinal tract, i.e., pH variations, gastric salts, ions, and enzymes. These interactions modify the composition of nanoparticles, leading to biomolecule adsorption and aggregation [ 75 , 76 , 77 ].
Although nanoparticles have toxic effects on the immune system and are involved in oxidative stress-related disorders, many people attribute these disorders to factors such as air pollution. This has led to proposals for public education on proper disposal of personal protective equipment in government-provided trash containers. Long-term monitoring of coastal waste and citizen initiatives for litter collection in populated areas have also been suggested [ 78 , 79 , 80 ], as has the recycling of carbon powders from masks for use in batteries [ 81 ] or renewable fuels [ 82 ], and the promotion of reusable alternatives and cellulose-fiber textiles. Potential disposal methods also include incineration and optimized pyrolysis [ 82 , 83 ].
The toxicity of metal nanoparticles varies according to the size, surficial coating, and shape of the nanoparticles [ 84 ]. The solubility of AgNPs is inversely proportional to the size of the nanoparticle. Due to increased dissolution and cell penetration efficacy, small nanoparticles exhibit high toxicity, with a strong attachment to DNA also causing DNA damage. Use AgNPs sized more than 20 nm shows less genotoxicity [ 85 , 86 ]. As metal nanoparticles sized less than 100 nm cause increased toxicity in vivo, the recommended ranges lie between 100 and 150 nm [ 87 , 88 ].
Toxicity is also influenced by the type of nanoparticle coating. Coating AgNPs with polyvinylpyrrolidone (PVP) has been found to have a greater toxicity and tissue uptake than citrate coatings, while positively charged polymers such as chitosan enhance the toxicity of AgNPs more than citrate-stabilized particles do. A bovine serum albumin (BSA) coating of gold nanoparticles (AuNPs) also leads to greater toxicity and poorer renal clearance than a glutathione (GSH) coating. On the other hand, coating AuNPs with PEG reduces nanoparticle toxicity and appears to be a suitable coating option [ 85 , 89 , 90 , 91 , 92 ].
Nanoparticles in vaccines and risk assessment
The WHO defines vaccines as pharmaceutical formulations that activate the immune system in order to produce specific antibodies, thereby generating protective immunity against a disease caused by a pathogen [ 93 ]. The conventional vaccines developed since the late eighteenth century rely on the discovery of antibodies in patients who have recovered from infections. To elicit an immune response, these use attenuated or inactivated pathogens and purified pathogen fragments [ 94 ]. Second-generation vaccines are produced using recombinant DNA technology in bacteria or in cell cultures [ 95 ].
Recently, a third generation of vaccines has emerged, which introduces the gene encoding the protective antigen into a host cell. By improving antigen processing and its presentation to antigen-presenting cells (APCs), this enhances the activation of CD4 + and CD8 + cells. This recent advance in vaccine technology holds promise for eliciting protective immune responses against the virus [ 96 ].
To overcome the limitations of conventional vaccines, i.e., attenuated or inactivated viruses, alternative options such as RNA- or DNA-based vaccines have also been sought recently. These new RNA- or DNA-based vaccine production technologies aim both to improve reactivity and efficacy and to reduce the cost of vaccines. They can also be used to effectively treat other diseases, such as cancer. But whereas vaccines need to be delivered to the right places in the body in a suitable form so as to prepare the immune system to combat an invading pathogen effectively, most vaccine molecules are prone to degradation. Due to limited accessibility and poor cell permeation [ 97 ], they may not be recognized efficiently by the immune system. A crucial role in enhancing the effectiveness of vaccines is played by delivery systems based on nanoformulations. By tailoring nanoencapsulation, vaccines can be delivered with precision and stability [ 96 ].
These delivery systems contribute to the in vivo behavior of vaccines in various ways: they protect vaccines from enzymatic degradation, improve their pharmacokinetic properties through surface engineering techniques such as PEGylation, enable active targeting to specific organs or cell types, and engineer controlled release of vaccines [ 93 , 98 , 99 ].
Lipid nanoparticles, self-assembling protein nanoparticles, virus-like particles, liposomes, and cationic nanoemulsion vaccines have been designed against SARS-CoV-2 [ 100 ]. The most prominent vaccines against SARS-CoV-2 are lipid-based nanoparticles (LNPs), which have been designed as drug nanocarriers for nucleic acid delivery [ 101 ]. By protecting fragile and unstable nucleic acids from degradation by nucleases, LNPs can increase the half-life of nucleic acids in the blood circulation. Charge-reversible LNPs contain ionizable lipids, either positively or negatively charged, that allow the LNPs to remain neutrally charged in the bloodstream, effective encapsulation of nucleic acids in the LNPs, and a high degree of endosomal escape of LNPs (Fig. 1 ) [ 102 ].
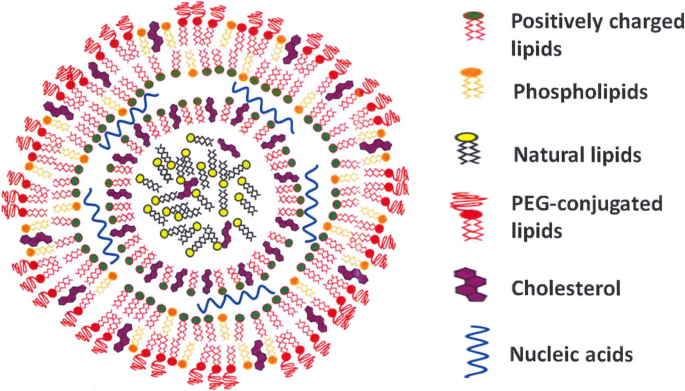
Schematic illustration of an mRNA-based SARS-CoV-2 lipid nanoparticle vaccine. Different components of the vaccine are visualized, i.e., positively charged lipids, phospholipids, natural lipids, PEGylated lipids, cholesterol, and nucleic acids. This figure is modified from those published in [ 105 ]. PEG, polyethylene glycol
LNPs contain two other main components: cholesterol, a neutral phospholipid, and PEG-lipid, which protects LNPs from phagocytosis and aggregation in the blood circulation and also during manufacturing and storage. In vaccine formulations, the PEG-lipid also ensures that the LNP maintains the desired diameter (200 nm) [ 103 ]. A factor that is crucial to efficient nucleic acid delivery is the complete escape of nucleic acids from the endosomal compartment after LNP internalization. By increasing the diffusibility of PEG-lipids, the addition of distearoylphosphatidylcholine (DSPC) and dioleoylphosphatidylethanolamine (DOPE) lipids to nanoparticles enhances endosomal escape [ 104 ].
To produce viral proteins, leading anti-COVID vaccine developers such as Moderna, Pfizer/BioNTech, CureVac, Walvax, Sanofi, Pasteur, and Entos Pharmaceuticals all use cationic LNPs to deliver mRNA or DNA encapsulated into host cells. Although mRNA vaccines are more prone to instability and functional defects than DNA vaccines, they are preferred due to their higher immunogenicity, their direct translation in the cytosol, and their higher loading potential into LNPs [ 106 , 107 , 108 ]. To achieve the same level of efficiency, self-amplifying mRNA-LNP vaccines such as those developed by Imperial College London and Arcturus/Duke-NUS require ten times less mRNA than mRNA vaccines. However, they have less flexibility in nucleotide modification than their mRNA counterparts [ 102 , 109 ].
Most LNP-derived vaccines currently available induce immune responses against the Spike protein (S protein). Interestingly, the receptor binding (RBD) and N-terminal (NTD) domains of the S protein are targeted by the most potent of the 61 monoclonal antibodies isolated from infected patients [ 110 ]. As anti-NTD antibodies inhibit and anti-RBD antibodies neutralize viral infections [ 111 ], the presentation of one of the virus protein domains is preferred above presentation of the whole protein for optimal immunity against new COVID-19 variants.
Due to the need for expensive low-temperature storage required by the SARS-CoV-2 vaccines currently available, their distribution poses challenges in developing countries. As the mechanical stresses caused by shaking might lead to aggregation and mRNA degradation in LNPs, vaccines also need to be administered promptly after preparation [ 112 , 113 , 114 ]. By enhancing the long-term stability of mRNA-LNPs, freeze-drying offers a solution to both these problems. However, if freeze-drying is to be successful, vaccine structure should not be affected by the lyoprotectants and by temperature stress. A new generation of the Pfizer/BioNTech vaccine is currently being prepared in lyophilized (freeze-dried) form [ 115 ].
Adverse effects associated with SARS-CoV-2 nanoparticle vaccines
Mild to moderate side effects are experienced after vaccinations. Compared to conventional vaccines, Pfizer and Moderna vaccines have been shown to cause more serious allergic reactions, including anaphylaxis. While side effects such as flushing and transient dyspnea were also observed in some of these mRNA vaccines, they were not considered to be allergic reactions [ 116 ]. Similar side effects were reported in earlier clinical safety studies of mRNA vaccines against influenza [ 116 ].
Although the rate of allergic reactions for LNP vaccines containing mRNA cargo is generally around 1.31 (95% CI, 0.90–1.84) per million doses, the number of severe immune reactions may be higher with booster doses [ 117 ]. LNPs induce inflammation, especially in non-adherent cells, due to the higher availability of cell surface receptors than in adherent cells [ 118 , 119 ]. The main suspect for anaphylactic reactions in mRNA vaccines is the coating polymer, PEG, which alters the water solubility of the vaccine-containing nanoparticles [ 120 ]. Although PEG is widely used in cosmetics, food, medication, and pharmaceutical agents, its use in vaccine technology is rare [ 121 ].
Initially, PEG molecules were thought to be safe and biologically inert, but nowadays PEG and PEG-like polymers are not considered to be as safe as initially thought [ 122 ]. An immune response mediated by anti-PEG IgG antibodies may develop in allergic individuals, particularly females [ 123 ]. These antibodies can target the PEG backbone or specifically bind to PEG terminal functional groups [ 124 ]. In the presence of reactive oxygen species, anti-PEG antibodies detrimentally affect the respiratory chain and signal transduction pathways, and also disrupt cell membranes [ 125 ]. In vivo, oxidation of PEG, especially of the PEG low-molecular polymer chains, produces toxic molecules, i.e., glycolic acid and hydroxy acid metabolites [ 126 ]. PEGylated nanoparticles cause pseudoallergic reactions such as complement-activation-related pseudo allergy (CARPA) [ 127 ] and toxic or immunogenic responses, particularly with booster doses. Anaphylactic responses to PEG occur in 2–8 cases per year worldwide, which has led the clinical use of two PEGylated pharmaceuticals to be abandoned [ 128 , 129 , 130 ]. The concentration of PEG in mRNA vaccines is much lower than in PEGylated drugs, and intramuscular administration induces less inflammation [ 131 ]. While anaphylactic reactions are caused not only by PEG, allergic reactions are also caused by vaccine components such as polysorbate 80 in the vaccines developed by AstraZeneca and Johnson [ 132 ]. On the other hand, polysorbate 80 is considered to be safer than PEG [ 128 ]. Figure 2 provides a schematic illustration of the various SARS-CoV-2 vaccines, and Table 3 a summary of the side effects associated with them.
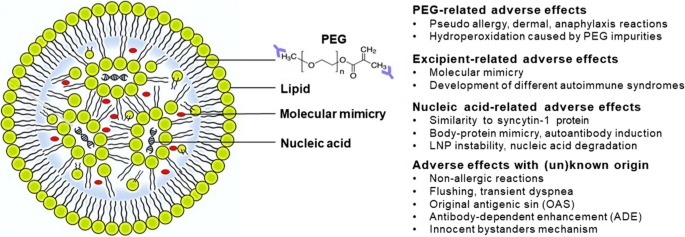
Categorized adverse effects associated with different components of nucleic acid-based lipid nanoparticle vaccines. PEG, excipient, or nucleic-acid-related adverse effects, and adverse effects of known and unknown origin are indicated. Formation of anti-PEG backbone auto-antibodies (blue). PEG, polyethylene glycol
The side effects of PEGylated vaccines and polysorbate-containing vaccines include urticaria, dizziness, diarrhea, wheezing, and tachycardia [ 133 ]. Dermal side effects, such as erythema or swelling, are slightly more common with mRNA vaccines than with adenoviral vaccines (10–15% versus 5–7% of the patients) [ 134 , 135 , 136 ]. Rare side effects of viral vector vaccines include thrombosis and thrombocytopenia. When there is cross-reactivity between PEG and polysorbates, immediate hypersensitivity reactions occur [ 137 , 138 ]. For approximately 6 months, mRNA and adenoviral vaccines can both cause changes in menstrual cycles, such as dysmenorrhea, alterations in frequency, volume, or cessation of bleeding. Women with pre-existing platelet disorders [ 139 ], those taking estrogen-based contraceptives [ 140 ], and those with thrombocytopenia [ 141 ] are all at a higher risk for such changes in their menstrual cycle. Messenger RNA- and viral vaccines affect the menstrual cycle, but the strongest changes are observed with mRNA-LNP vaccines [ 142 , 143 ].
A major concern with the use of nanoparticle vaccines is that they trigger autoimmune diseases. SARS-CoV-2 mRNA-NPs vaccines trigger autoimmune liver diseases, Guillain-Barré syndrome, IgA nephropathy, myocarditis, optical neuromyelitis, autoimmune polyarthritis, Graves’ disease, type 1 diabetes mellitus, and systemic lupus erythematosus [ 144 , 145 , 146 , 147 , 148 , 149 ]. A second concern is the possibility of reverse transcription of mRNA vaccines in liver cells, which has been observed in vitro [ 150 ], although genotoxicity in vivo remains debatable [ 151 ]. A third concern is the phenomenon of original antigenic sin (OAS), which occurs when antibodies from previous infections or vaccinations hinder the neutralization of newly mutated antigens, particularly the omicron antigen variant [ 152 ]. A fourth concern is the concept of antibody-dependent enhancement (ADE), where low antibody titers bind to virus particles without neutralizing them, thereby facilitating virus entry into macrophages and enhancing respiratory disease responses. ADE has been linked to SARS-CoV-2 mRNA-NP vaccines [ 153 ].
A fifth concern has also arisen regarding the cross-reaction of vaccine-induced antibodies against syncytin-1, a placental protein similar to the SARS-CoV-2 spike protein, which activates the immune system and impacts female pregnancy [ 154 ]. Another significant consequence that can arise, mainly in young men, after a second dose of mRNA-based vaccines is myocarditis; this has an incidence of 12.6 cases per million. Sex hormones can contribute to the development of myocarditis [ 155 ], which is not only related to molecular mimicry of S protein, self-antigens, or the formation of autoantibodies, but may also be caused by vaccine adjuvants, activation of “innocent bystanders,” or induction of autoantibodies [ 149 ].
A question we are currently unable to answer is whether the S protein should be replaced by other viral proteins as the immunogenic target to develop vaccines. New generations of vaccines such as spike-trimers and spike-ferritin in liposomes will continue to be based on spike proteins [ 156 , 157 ]. To address and mitigate the side effects of nanoparticle-based vaccines, certain modifications might be considered. PEG chain length and topological configuration affect immunogenicity. Pre-treatment with small amounts of high-molecular-weight PEG reduces anti-PEG reactions, and in animal models, short and hyperbranched PEG polymers, such as poly(oligo-ethylene glycol) methacrylate, exhibit decreased interaction with anti-PEG IgG and IgM antibodies [ 158 ]. To prevent inflammation and side effects, glyceryl monostearate (GMS) should not be included in LNPs [ 118 ]. Promising alternatives for PEG are polyglycerol polyricinoleate, polysarcosine, polyhydroxypropylmethacrylamide, polysulfobetaine, and polycarboxybetaine polymers [ 159 ]. Replacing PEG with polysulfobetaine coating results in higher biological activity of insulin; in nude mice, a dextran coating eliminated toxicity and liver stress of iron oxide nanoparticles [ 160 , 161 ]. Polyesters like polycarbonates and polyphosphoesters might also be viable alternatives to PEG, since they degrade in vivo into non-toxic fragments, and can be easily produced using ring-opening polymerization (ROP) [ 162 ].
More preclinical studies are needed to evaluate vaccines. As two-dimensional in vitro studies may not always completely capture the complex immune environment, and as the phenotype and expression of receptors on cells may be influenced by culture conditions [ 163 ], the prediction of vaccine performance systems might be improved by three-dimensional cell culture systems [ 164 ] and/or standardized in vitro culture systems [ 164 ]. And as small rodents are anatomically different from humans, non-human primates might be more reliable for in vivo studies [ 165 ].
When evaluating nucleic acid vaccines, it is essential to assess not only the quantitative distribution of DNA or mRNA cargo, but also protein expression. This will help to monitor the distribution, retention, and release pattern of the delivered DNA or mRNA, providing a predictive tool for vaccine safety. In addition, valuable insight into the tissue localization of delivered nanoparticles is provided by information on vaccine distribution in lymph nodes, organs, and APCs [ 100 ]. Although a skin-sensitization test is recommended before vaccination with PEG and polysorbate [ 121 , 128 , 166 , 167 ], the number of positive cases in skin tests is considerably lower than the number of sensitive cases after vaccine administration [ 168 ]. Protocols for graded dosing of vaccines have been developed for hypersensitive individuals, such as those with basophil disorders and uncontrolled asthma. Allergic individuals are advised to receive a second dose of a different vaccine, or, in some cases, heterologous prime-boost vaccines are recommended [ 169 , 170 , 171 ]. Finally, in Fig. 3 , we propose several solutions that will minimize the disadvantages associated with nanoparticle use.
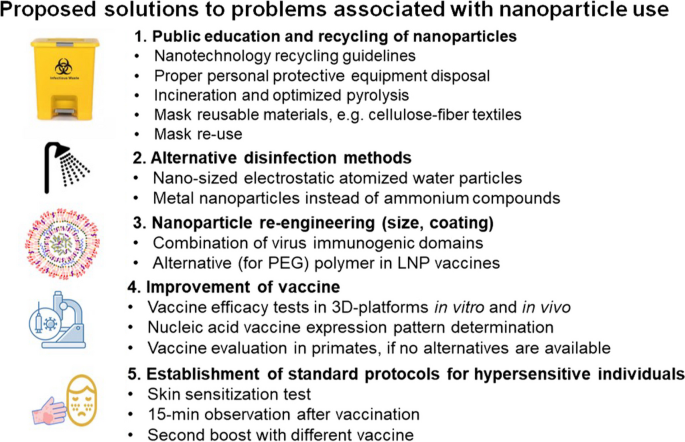
Proposed solutions for minimizing the disadvantages associated with nanoparticle usage
Conclusions
The use of nanoparticles in combatting viral infections has proved to represent a promising and valuable approach in the realm of global health. The COVID-19 pandemic underscored the significance of nanotechnology in vaccine development, infection prevention, and therapeutic strategies. By offering innovative solutions — including functionalized face masks, antiviral therapeutics, and diagnostic platforms — nanoparticles have already showcased their potential in pandemic control. However, the potential risks and challenges associated with their use still require attention, particularly in vaccine development.
Existing LNP formulations have played a crucial role in the rapid development of SARS-CoV-2 vaccines. Unfortunately, vaccine-induced immunity against SARS-CoV-2 is of limited duration, and, to enhance vaccine safety, adverse effects such as anaphylaxis and autoimmune reactions call for modifications in nanoparticle design such as after receiving primary doses, individuals with a history of COVID-19 vaccine anaphylaxis should not receive booster doses of the same vaccine.
The development of long-lasting and immunogenic nanoparticle formulations against SARS-CoV-2 is crucial. To prevent nanoparticle aggregation, surface modification of LNPs is also vital. It is also possible that alternative coating materials, such as shorter-length PEG polymers or other synthetic or natural polymers, may help to minimize side effects and enhance vaccine safety.
Whatever their promise in combating viral infections, the main concerns raised by metal nanoparticles involve their entry into the ecosystem. However, they can also aid in wastewater treatment, microplastic degradation, and environmentally friendly H 2 O and CO 2 production. If responsible nanoparticle use is to be ensured, it is imperative to achieve better control of their toxicity through modifications of nanoparticle size, surface coating, and shape, and also to stimulate public education and proper disposal practices for nanoparticle-based personal protective equipment. Although multiple factors determine whether nanoparticles are toxic, very little information is available on their toxicity, which is sometimes related to the specific drug delivery, and/or to the physical characteristics of the nanoparticles (i.e., their size, surface area, charge, shape, and composition). The adverse effects associated with the use of nanoparticles such as LNPs containing PEG (PEGylated LNPs; Fig. 2 ) limit the use of LNPs in clinical applications. PEG is an FDA-approved compound that is used in pharmacochemical and personal care products. A solution to problems involving its toxicity in clinical uses may be provided by LNP modification, such as by replacing PEG with natural polymers.
The lessons learned from the COVID-19 pandemic have shed light on the importance of responsible and ethical nanoparticle use. In the pursuit of future pandemic control and global health protection, it is essential to continue harnessing the potential of nanotechnology while simultaneously remaining cautious and well-informed. By prioritizing the safety of nanoparticle-based products and vaccines, we will be able to ensure that nanotechnology remains a valuable tool in our fight against viral outbreaks, with minimized risks and enhanced benefits for both human health and the environment.
Kausar S, Said Khan F, Ishaq Mujeeb Ur Rehman M, Akram M, Riaz M, Rasool G, Hamid Khan A, Saleem I, Shamim S, Malik A (2021) A review: mechanism of action of antiviral drugs. Int J Immunopathol Pharmacol 35:731–738. https://doi.org/10.1177/2F20587384211002621
Article Google Scholar
Figueiró Longo JP, Muehlmann LA (2021) How has nanomedical innovation contributed to the COVID-19 vaccine development? Nanomedicine (Lond) 16:731–738. https://doi.org/10.2217/nnm-2021-0035
Article CAS Google Scholar
Chen L, Liang J (2020) An overview of functional nanoparticles as novel emerging antiviral therapeutic agents. Mater Sci Eng C 112:110924. https://doi.org/10.1016/j.msec.2020.110924
Mallapaty S (2021) Laos bats host closest known relatives of virus behind COVID. Nature 597:603. https://doi.org/10.1038/d41586-021-02596-2
Sportelli MC, Izzi M, Kukushkina EA, Hossain SI, Picca RA, Ditaranto N, Cioffi N (2020) Can nanotechnology and materials science help the fight against SARS-CoV-2? Nanomaterials 10:731–738. https://doi.org/10.3390/nano10040802
Silva LF, Dotto GL, Pinto D, Oliveira ML (2021) Nanoparticles and interfaces with toxic elements in fluvial suspended sediment. Mar Pollut Bull 168:112405. https://doi.org/10.1016/j.marpolbul.2021.112405
Oliveira ML, Dotto GL, Pinto D, Neckel A, Silva LF (2021) Nanoparticles as vectors of other contaminants in estuarine suspended sediments: natural and real conditions. Mar Pollut Bull 168:112429. https://doi.org/10.1016/j.marpolbul.2021.112429
López AF, Fabiani M, Lassalle V, Spetter C, Severini MF (2022) Critical review of the characteristics, interactions, and toxicity of micro/nanomaterials pollutants in aquatic environments. Mar Pollut Bull 174:731–738. https://doi.org/10.1016/j.marpolbul.2021.113276
Krantz MS, Phillips EJ (2022) COVID-19 mRNA vaccine safety during the first 6 months of roll-out in the USA. Lancet Infect Dis 35:731–738. https://doi.org/10.1016/S1473-3099(22)00123-2
De-la-Torre GE, Pizarro-Ortega CI, Dioses-Salinas DC, Ammendolia J, Okoffo ED (2021) Investigating the current status of COVID-19 related plastics and their potential impact on human health. Curr Opin Toxicol 35:731–738. https://doi.org/10.1016/j.cotox.2021.08.002
Campos EV, Pereira AE, De Oliveira JL, Carvalho LB, Guilger-Casagrande M, De Lima R, Fraceto LF (2020) How can nanotechnology help to combat COVID-19? Opportunities and urgent need. J Nanobiotechnology 18:1–23. https://doi.org/10.1186/s12951-020-00685-4
Seidi F, Deng C, Zhong Y, Liu Y, Huang Y, Li C, Xiao H (2021) Functionalized masks: powerful materials against COVID-19 and future pandemics. Small 17:2102453. https://doi.org/10.1002/smll.202102453
Bradley D (2020) Copper against COVID. Mater Today (Kidlington, England) 40:2. https://doi.org/10.1016/j.mattod.2020.09.016
Skalny AV, Rink L, Ajsuvakova OP, Aschner M, Gritsenko VA, Alekseenko SI, Svistunov AA, Petrakis D, Spandidos DA, Aaseth J (2020) Zinc and respiratory tract infections: perspectives for COVID-19. Int J Mol Med 46:17–26. https://doi.org/10.3892/ijmm.2020.4575
Sousa BC, Cote DL (2020) Antimicrobial copper cold spray coatings and SARS-CoV-2 surface inactivation. MRS Adv 5:2873–2880. https://doi.org/10.1557/adv.2020.366
Joost U, Juganson K, Visnapuu M, Mortimer M, Kahru A, Nõmmiste E, Kisand V, Ivask A (2015) Photocatalytic antibacterial activity of nano-TiO 2 (anatase)-based thin films: effects on Escherichia coli cells and fatty acids. J Photochem Photobiol B Biol 142:178–185. https://doi.org/10.1016/j.jphotobiol.2014.12.010
Xiao MF, Zeng C, Li SH, Yuan FL (2021) Applications of nanomaterials in COVID-19 pandemic. Rare Met 41:1–13. https://doi.org/10.1007/s12598-021-01789-y
Bhattacharjee S, Joshi R, Chughtai AA, Macintyre CR (2019) Graphene modified multifunctional personal protective clothing. Adv Mater Interfaces 6:1900622. https://doi.org/10.1002/admi.201900622
Palmieri V, De Maio F, De Spirito M, Papi M (2021) Face masks and nanotechnology: keep the blue side up. Nano Today 37:101077. https://doi.org/10.1016/j.nantod.2021.101077
El-Atab N, Qaiser N, Badghaish H, Shaikh SF, Hussain MM (2020) Flexible nanoporous template for the design and development of reusable anti-COVID-19 hydrophobic face masks. ACS Nano 14:7659–7665. https://doi.org/10.1021/acsnano.0c03976
Choi S, Jeon H, Jang M, Kim H, Shin G, Koo JM, Lee M, Sung HK, Eom Y, Yang HS (2021) Biodegradable, efficient, and breathable multi-use face mask filter. Adv Sci 8:2003155. https://doi.org/10.1002/advs.202003155
Almanza-Reyes H, Moreno S, Plascencia-López I, Alvarado-Vera M, Patrón-Romero L, Borrego B, Reyes-Escamilla A, Valencia-Manzo D, Brun A, Pestryakov A (2021) Evaluation of silver nanoparticles for the prevention of SARS-CoV-2 infection in health workers: in vitro and in vivo. Plos One 16:e0256401. https://doi.org/10.1371/journal.pone.0256401
ClinicalTrials.gov (2021) Evaluation of silver nanoparticles for the prevention of COVID-19 (COVID-19). (NCT04894409). https://clinicaltrials.gov/ct2/show/NCT04894409
Talebian S, Wallace GG, Schroeder A, Stellacci F, Conde J (2020) Nanotechnology-based disinfectants and sensors for SARS-CoV-2. Nat Nanotechnol 15:618–621. https://doi.org/10.1038/s41565-020-0751-0
Yasugi M, Komura Y, Ishigami Y (2022) Mechanisms underlying inactivation of SARS-CoV-2 by nano-sized electrostatic atomized water particles. J Nanopart Res 24:99. https://doi.org/10.1007/s11051-022-05485-5
Hora PI, Pati SG, McNamara PJ, Arnold WA (2020) Increased use of quaternary ammonium compounds during the SARS-CoV-2 pandemic and beyond: consideration of environmental implications. Environ Sci Technol Lett 7:622–631. https://doi.org/10.1021/acs.estlett.0c00437
Riva L, Yuan S, Yin X, Martin-Sancho L, Matsunaga N, Pache L, Burgstaller-Muehlbacher S, De Jesus PD, Teriete P, Hull MV (2020) Discovery of SARS-CoV-2 antiviral drugs through large-scale compound repurposing. Nature 586:113–119. https://doi.org/10.1038/s41586-020-2577-1
De Clercq E (2006) Antiviral agents active against influenza A viruses. Nat Rev Drug Discov 5:1015–1025. https://doi.org/10.1038/nrd2175
Abo-Zeid Y, Ismail NS, McLean GR, Hamdy NM (2020) A molecular docking study repurposes FDA approved iron oxide nanoparticles to treat and control COVID-19 infection. Eur J Pharm Sci 153:105465. https://doi.org/10.1016/j.ejps.2020.105465
Behbahanipour M, Benoit R, Navarro S, Ventura S (2023) Oligobinders: bioengineered soluble amyloid-like nanoparticles to bind and neutralize SARS-CoV-2. ACS Appl Mater Interfaces 15:11444–11457. https://doi.org/10.1021/acsami.2c18305
Page TM, Nie C, Neander L, Povolotsky TL, Sahoo AK, Nickl P, Adler JM, Bawadkji O, Radnik J, Achazi K (2023) Functionalized fullerene for inhibition of SARS-CoV-2 variants. Small 19:2206154. https://doi.org/10.1002/smll.202206154
Li Z, Wang Z, Dinh P-UC, Zhu D, Popowski KD, Lutz H, Hu S, Lewis MG, Cook A, Andersen H (2021) Cell-mimicking nanodecoys neutralize SARS-CoV-2 and mitigate lung injury in a non-human primate model of COVID-19. Nat Nanotechnol 16:942–951. https://doi.org/10.1038/s41565-021-00923-2
Milovanovic M, Arsenijevic A, Milovanovic J, Kanjevac T, Arsenijevic N (2017) Nanoparticles in antiviral therapy. In: Antimicrobial nanoarchitectonics. Elsevier, pp 383–410. https://doi.org/10.1016/B978-0-323-52733-0.00014-8
Sanna V, Satta S, Hsiai T, Sechi M (2022) Development of targeted nanoparticles loaded with antiviral drugs for SARS-CoV-2 inhibition. Eur J Med Chem 231:114121. https://doi.org/10.1016/j.ejmech.2022.114121
Khater SE, El-Khouly A, Abdel-Bar HM, Al-Mahallawi AM, Ghorab DM (2021) Fluoxetine hydrochloride loaded lipid polymer hybrid nanoparticles showed possible efficiency against SARS-CoV-2 infection. Int J Pharm 607:121023. https://doi.org/10.1016/j.ijpharm.2021.121023
Naseem T, Durrani T (2021) The role of some important metal oxide nanoparticles for wastewater and antibacterial applications: a review. Environ Chem Ecotoxicol 3:59–75. https://doi.org/10.1016/j.enceco.2020.12.001
Uheida A, Mejía HG, Abdel-Rehim M, Hamd W, Dutta J (2021) Visible light photocatalytic degradation of polypropylene microplastics in a continuous water flow system. J Hazard Mater 406:124299. https://doi.org/10.1016/j.jhazmat.2020.124299
Hydzik P (2012) Nanoparticles toxicity--selective examples. Przegl Lek 69:486–489. PMID: 23243914 https://europepmc.org/article/med/23243914
Shirvanimoghaddam K, Czech B, Yadav R, Gokce C, Fusco L, Delogu LG, Yilmazer A, Brodie G, Al-Othman AK, Al-Tamimi AK (2022) Facemask global challenges: the case of effective synthesis, utilization, and environmental sustainability. Sustainability 14:737. https://doi.org/10.3390/su14020737
Vishwakarma K, Upadhyay N, Singh J, Liu S, Singh VP, Prasad SM, Chauhan DK, Tripathi DK, Sharma S (2017) Differential phytotoxic impact of plant-mediated silver nanoparticles (AgNPs) and silver nitrate (AgNO3) on Brassica sp. Front Plant Sci 8:1501. https://doi.org/10.1016/j.aquatox.2016.04.019
Dietz K-J, Herth S (2011) Plant nanotoxicology. Trends Plant Sci 16:582–589. https://doi.org/10.1016/j.tplants.2011.08.003
Begum P, Fugetsu B (2012) Phytotoxicity of multi-walled carbon nanotubes on red spinach (Amaranthus tricolor L) and the role of ascorbic acid as an antioxidant. J Hazard Mater 243:212–222. https://doi.org/10.1016/j.jhazmat.2012.10.025
Slomberg DL, Schoenfisch MH (2012) Silica nanoparticle phytotoxicity to Arabidopsis thaliana. Environ Sci Technol 46:10247–10254. https://doi.org/10.1021/es300949f
Khan M, Khan MSA, Borah KK, Goswami Y, Hakeem KR, Chakrabartty I (2021) The potential exposure and hazards of metal-based nanoparticles on plants and environment, with special emphasis on ZnO NPs, TiO 2 NPs, and AgNPs: a review. Environ Adv 6:100128. https://doi.org/10.1016/j.envadv.2021.100128
Dağhan H (2018) Effects of TiO2 nanoparticles on maize (Zea mays L.) growth, chlorophyll content, and nutrient uptake. Appl Ecol Environ Res 16:6873–6883. https://doi.org/10.15666/aeer/1605_68736883
Lin D, Xing B (2008) Root uptake and phytotoxicity of ZnO nanoparticles. Environ Sci Technol 42:5580–5585. https://doi.org/10.1021/es800422x
Monica RC, Cremonini R (2009) Nanoparticles and higher plants. Caryologia 62:161–165. https://doi.org/10.1080/00087114.2004.10589681
Raskar S, Laware S (2014) Effect of zinc oxide nanoparticles on cytology and seed germination in onion. Int J Curr Microbiol App Sci 3:467–473. https://www.ijcmas.com/vol-3-2/S.V.Raskar%20and%20S.L.Laware.pdf
Li CC, Wang YJ, Dang F, Zhou DM (2016) Mechanistic understanding of reduced AgNP phytotoxicity induced by extracellular polymeric substances. J Hazard Mater 308:21–28. https://doi.org/10.1016/j.jhazmat.2016.01.036
Wang C, Jiang K, Wu B, Zhou J, Lv Y (2018) Silver nanoparticles with different particle sizes enhance the allelopathic effects of Canada goldenrod on the seed germination and seedling development of lettuce. Ecotoxicology 27:1116–1125. https://doi.org/10.1007/s10646-018-1966-9
Federici G, Shaw BJ, Handy RD (2007) Toxicity of titanium dioxide nanoparticles to rainbow trout (Oncorhynchus mykiss): gill injury, oxidative stress, and other physiological effects. Aquat Toxicol 84:415–430. https://doi.org/10.1016/j.aquatox.2007.07.009
Huang CW, Li SW, Liao VH-C (2017) Chronic ZnO-NPs exposure at environmentally relevant concentrations results in metabolic and locomotive toxicities in Caenorhabditis elegans. Environ Pollut 220:1456–1464. https://doi.org/10.1016/j.envpol.2016.10.086
Bundschuh M, Filser J, Lüderwald S, McKee MS, Metreveli G, Schaumann GE, Schulz R, Wagner S (2018) Nanoparticles in the environment: where do we come from, where do we go to? Environ Sci Europe 30:1–17. https://doi.org/10.1186/s12302-018-0132-6
Labille J, Slomberg D, Catalano R, Robert S, Apers-Tremelo M-L, Boudenne J-L, Manasfi T, Radakovitch O (2020) Assessing UV filter inputs into beach waters during recreational activity: a field study of three French Mediterranean beaches from consumer survey to water analysis. Sci Total Environ 706:136010. https://doi.org/10.1016/j.scitotenv.2019.136010
Wang J, Zhu X, Zhang X, Zhao Z, Liu H, George R, Wilson-Rawls J, Chang Y, Chen Y (2011) Disruption of zebrafish (Danio rerio) reproduction upon chronic exposure to TiO 2 nanoparticles. Chemosphere 83:461–467. https://doi.org/10.1016/j.chemosphere.2010.12.069
Bodamyali T, Stevens CR, Blake DR, Winyard PG (2000) Reactive oxygen/nitrogen species and acute inflammation: a physiological process. In: Free Radicals and Inflammation. Springer, pp 11–16. https://doi.org/10.1007/978-3-0348-8482-2_2
Jakubczyk K, Dec K, Kałduńska J, Kawczuga D, Kochman J, Janda K (2020) Reactive oxygen species-sources, functions, oxidative damage. Pol Merkur Lekarski 48:124–127
Google Scholar
Valko M, Leibfritz D, Moncol J, Cronin MTD, Mazur M, Telser J (2007) Free radicals and antioxidants in normal physiological functions and human disease. Int J Biochem Cell Biol 39:44–84. https://doi.org/10.1016/j.biocel.2006.07.001
Natarajan P, Tomich JM (2020) Understanding the influence of experimental factors on bio-interactions of nanoparticles: towards improving correlation between in vitro and in vivo studies. Arch Biochem Biophys 694:108592. https://doi.org/10.1016/j.abb.2020.108592
Sohal IS, Cho YK, O’Fallon KS, Gaines P, Demokritou P, Bello D (2018) Dissolution behavior and biodurability of ingested engineered nanomaterials in the gastrointestinal environment. ACS Nano 12:8115–8128. https://doi.org/10.1021/acsnano.8b02978
De Matteis V (2017) Exposure to inorganic nanoparticles: routes of entry, immune response, biodistribution and in vitro/in vivo toxicity evaluation. Toxics 5:29. https://doi.org/10.3390/toxics5040029
Yang L, Ji W, Mao M, Huang J-n (2020) An updated review on the properties, fabrication and application of hybrid-nanofluids along with their environmental effects. J Clean Prod 257:120408. https://doi.org/10.1016/j.jclepro.2020.120408
Hsin Y-H, Chen C-F, Huang S, Shih T-S, Lai P-S, Chueh PJ (2008) The apoptotic effect of nanosilver is mediated by a ROS-and JNK-dependent mechanism involving the mitochondrial pathway in NIH3T3 cells. Toxicol Lett 179:130–139. https://doi.org/10.1016/j.toxlet.2008.04.015
ATSDR (2014) Agency for Toxic Substances and Disease Registry (ATSDR), Toxicological profile for silver. https://wwwn.cdc.gov/TSP/ToxProfiles/ToxProfiles.aspx?id=539&tid=97 . Accessed 26 March 2014
Rezvani E, Rafferty A, McGuinness C, Kennedy J (2019) Adverse effects of nanosilver on human health and the environment. Acta Biomater 94:145–159. https://doi.org/10.1016/j.actbio.2019.05.042
Kiss B, Bíró T, Czifra G, Tóth BI, Kertész Z, Szikszai Z, Kiss ÁZ, Juhász I, Zouboulis CC, Hunyadi J (2008) Investigation of micronized titanium dioxide penetration in human skin xenografts and its effect on cellular functions of human skin-derived cells. Exp Dermatol 17:659–667. https://doi.org/10.1111/j.1600-0625.2007.00683.x
Lademann J, Weigmann H-J, Rickmeyer C, Barthelmes H, Schaefer H, Mueller G, Sterry W (1999) Penetration of titanium dioxide microparticles in a sunscreen formulation into the horny layer and the follicular orifice. Skin Pharmacol Physiol 12:247–256. https://doi.org/10.1159/000066249
Bennat C, Müller-Goymann C (2000) Skin penetration and stabilization of formulations containing microfine titanium dioxide as physical UV filter. Int J Cosmet Sci 22:271–283. https://doi.org/10.1046/j.1467-2494.2000.00009.x
Mavon A, Miquel C, Lejeune O, Payre B, Moretto P (2007) In vitro percutaneous absorption and in vivo stratum corneum distribution of an organic and a mineral sunscreen. Skin Pharmacol Physiol 20:10–20. https://doi.org/10.1159/000096167
Christensen FM, Johnston HJ, Stone V, Aitken RJ, Hankin S, Peters S, Aschberger K (2011) Nano-TiO 2 –feasibility and challenges for human health risk assessment based on open literature. Nanotoxicology 5:110–124. https://doi.org/10.3109/17435390.2010.504899
Oberdörster G, Sharp Z, Atudorei V, Elder A, Gelein R, Kreyling W, Cox C (2004) Translocation of inhaled ultrafine particles to the brain. Inhal Toxicol 16:437–445. https://doi.org/10.1080/08958370490439597
Gutwein LG, Webster TJ (2004) Increased viable osteoblast density in the presence of nanophase compared to conventional alumina and titania particles. Biomaterials 25:4175–4183. https://doi.org/10.1016/j.biomaterials.2003.10.090
Pollard ZA, Karod M, Goldfarb JL (2021) Metal leaching from antimicrobial cloth face masks intended to slow the spread of COVID-19. Sci Rep 11:19216. https://doi.org/10.1038/s41598-021-98577-6
Mercer RR, Scabilloni JF, Wang L, Battelli LA, Antonini JM, Roberts JR, Qian Y, Sisler JD, Castranova V, Porter DW (2018) The fate of inhaled nanoparticles: detection and measurement by enhanced dark-field microscopy. Toxicol Pathol 46:28–46. https://doi.org/10.1177/0192623317732321
Coreas R, Cao X, DeLoid GM, Demokritou P, Zhong W (2020) Lipid and protein corona of food-grade TiO2 nanoparticles in simulated gastrointestinal digestion. NanoImpact 20:100272. https://doi.org/10.1016/j.impact.2020.100272
Baimanov D, Wang J, Zhang J, Liu K, Cong Y, Shi X, Zhang X, Li Y, Li X, Qiao R (2022) In situ analysis of nanoparticle soft corona and dynamic evolution. Nat Commun 13:5389. https://doi.org/10.1038/s41467-022-33044-y
Zhou H, McClements DJ (2022) Recent advances in the gastrointestinal fate of organic and inorganic nanoparticles in foods. Nanomaterials 12:1099. https://doi.org/10.3390/nano12071099
Li L, Huang J, Almutairi AW, Lan X, Zheng L, Lin Y, Chen L, Fu N, Lin Z, Abomohra AE-F (2021) Integrated approach for enhanced bio-oil recovery from disposed face masks through co-hydrothermal liquefaction with Spirulina platensis grown in wastewater. Biomass Conv Bioref 13:11109–11120. https://doi.org/10.1007/s13399-021-01891-2
Li S, Ding J, Zheng X, Sui Y (2021) Beach tourists behavior and beach management strategy under the ongoing prevention and control of the COVID-19 pandemic: a case study of Qingdao. China Ocean Coast Manage 215:105974. https://doi.org/10.1016/j.ocecoaman.2021.105974
Hatami T, Rakib MRJ, Madadi R, De-la-Torre GE, Idris AM (2022) Personal protective equipment (PPE) pollution in the Caspian Sea, the largest enclosed inland water body in the world. Sci Total Environ 824:153771. https://doi.org/10.1016/j.scitotenv.2022.153771
Lee G, Lee ME, Kim SS, Joh H-I, Lee S (2022) Efficient upcycling of polypropylene-based waste disposable masks into hard carbons for anodes in sodium ion batteries. J Ind Eng Chem 105:268–277. https://doi.org/10.1016/j.jiec.2021.09.026
Zhao X, Klemeš JJ, You F (2022) Energy and environmental sustainability of waste personal protective equipment (PPE) treatment under COVID-19. Renew Sustain Energy Rev 153:111786. https://doi.org/10.1016/j.rser.2021.111786
Aragaw TA, Mekonnen BA (2021) Current plastics pollution threats due to COVID-19 and its possible mitigation techniques: a waste-to-energy conversion via pyrolysis. Environ Syst Res 10. https://doi.org/10.1186/s40068-020-00217-x
Sani A, Cao C, Cui D (2021) Toxicity of gold nanoparticles (AuNPs): a review. Biochem Biophys Rep 26:100991. https://doi.org/10.1016/j.bbrep.2021.100991
Kim K-T, Truong L, Wehmas L, Tanguay RL (2013) Silver nanoparticle toxicity in the embryonic zebrafish is governed by particle dispersion and ionic environment. Nanotechnology 24:115101. https://doi.org/10.1093/toxsci/kft081
Park MV, Neigh AM, Vermeulen JP, de la Fonteyne LJ, Verharen HW, Briedé JJ, van Loveren H, de Jong WH (2011) The effect of particle size on the cytotoxicity, inflammation, developmental toxicity, and genotoxicity of silver nanoparticles. Biomaterials 32:9810–9817. https://doi.org/10.1016/j.biomaterials.2011.08.085
Renwick L, Brown D, Clouter A, Donaldson K (2004) Increased inflammation and altered macrophage chemotactic responses caused by two ultrafine particle types. Occup Environ Med 61:442–447. https://doi.org/10.1136/oem.2003.008227
Sager TM, Kommineni C, Castranova V (2008) Pulmonary response to intratracheal instillation of ultrafine versus fine titanium dioxide: role of particle surface area. Part Fibre Toxicol 5:17. https://doi.org/10.1186/1743-8977-5-17
Hunt PR, Keltner Z, Gao X, Oldenburg SJ, Bushana P, Olejnik N, Sprando RL (2014) Bioactivity of nanosilver in Caenorhabditis elegans: effects of size, coat, and shape. Toxicol Rep 1:923–944. https://doi.org/10.1016/j.toxrep.2014.10.020
Nativo P, Prior IA, Brust M (2008) Uptake and intracellular fate of surface-modified gold nanoparticles. ACS Nano 2:1639–1644. https://doi.org/10.1021/nn800330a
Cruje C, Chithrani B (2015) Integration of peptides for enhanced uptake of pegylated gold nanoparticles. J Nanosci Nanotechnol 15:2125–2131. https://doi.org/10.1166/jnn.2015.10321
Zhang X-D, Wu D, Shen X, Liu P-X, Fan F-Y, Fan S-J (2012) In vivo renal clearance, biodistribution, toxicity of gold nanoclusters. Biomaterials 33:4628–4638. https://doi.org/10.1016/j.biomaterials.2012.03.020
Pollard AJ, Bijker EM (2021) A guide to vaccinology: from basic principles to new developments. Nat Rev Immunol 21:83–100. https://doi.org/10.1038/s41577-020-00497-5
Riedel S (2005) Edward Jenner and the history of smallpox and vaccination. Baylor University Med Center Proc 2005. Taylor & Francis. https://doi.org/10.1080/08998280.2005.11928028
Nascimento I, Leite L (2012) Recombinant vaccines and the development of new vaccine strategies. Braz J Med Biol Res 45:1102–1111. https://pubmed.ncbi.nlm.nih.gov/22948379/ , https://doi.org/10.1590/s0100-879x2012007500142
Lozano D, Larraga V, Vallet-Regí M, Manzano M (2023) An overview of the use of nanoparticles in vaccine development. Nanomaterials 13:1828. https://doi.org/10.3390/nano13121828
Delany I, Rappuoli R, De Gregorio E (2014) Vaccines for the 21st century. EMBO Mol Med 6:708–720. https://doi.org/10.1002/emmm.201403876
Kirtane AR, Verma M, Karandikar P, Furin J, Langer R, Traverso G (2021) Nanotechnology approaches for global infectious diseases. Nat Nanotechnol 16:369–384. https://doi.org/10.1038/s41565-021-00866-8
Gregory AE, Titball R, Williamson D (2013) Vaccine delivery using nanoparticles. Front Cell Infect Microbiol 3:13. https://doi.org/10.3389/fcimb.2013.00013
Lee J, Kim D, Byun J, Wu Y, Park J, Oh Y-K (2022) In vivo fate and intracellular trafficking of vaccine delivery systems. Adv Drug Deliv Rev 186:114325. https://doi.org/10.1016/j.addr.2022.114325
Akinc A, Maier MA, Manoharan M, Fitzgerald K, Jayaraman M, Barros S, Ansell S, Du X, Hope MJ, Madden TD (2019) The Onpattro story and the clinical translation of nanomedicines containing nucleic acid-based drugs. Nat Nanotechnol 14:1084–1087. https://doi.org/10.1038/s41565-019-0591-y
Buschmann MD, Carrasco MJ, Alishetty S, Paige M, Alameh MG, Weissman D (2021) Nanomaterial delivery systems for mRNA vaccines. Vaccines 9:65. https://doi.org/10.3390/vaccines9010065
Evers MJ, Kulkarni JA, van der Meel R, Cullis PR, Vader P, Schiffelers RM (2018) State-of-the-art design and rapid-mixing production techniques of lipid nanoparticles for nucleic acid delivery. Small Methods 2:1700375. https://doi.org/10.1002/smtd.201700375
Hou X, Zaks T, Langer R, Dong Y (2021) Lipid nanoparticles for mRNA delivery. Nat Rev Mater 7:65. https://doi.org/10.1038/s41578-021-00400-1
Kulkarni JA, Witzigmann D, Thomson SB, Chen S, Leavitt BR, Cullis PR, van der Meel R (2020) The current landscape of nucleic acid therapeutics. Nat Nanotechnol 15:963–963. https://doi.org/10.1038/s41565-021-00898-0
Klauer AA, van Hoof A (2012) Degradation of mRNAs that lack a stop codon: a decade of nonstop progress. Wiley Interdiscip Rev RNA 3:649–660. https://doi.org/10.1002/wrna.1124
Pardi N, Hogan MJ, Porter FW, Weissman D (2018) mRNA vaccines - a new era in vaccinology. Nat Rev Drug Discov 17:261–279. https://doi.org/10.1038/nrd.2017.243
Kulkarni JA, Witzigmann D, Thomson SB, Chen S, Leavitt BR, Cullis PR, van der Meel R (2021) The current landscape of nucleic acid therapeutics. Nat Nanotechnol 16:630–643. https://doi.org/10.1038/s41565-021-00898-0
Bloom K, van den Berg F, Arbuthnot P (2021) Self-amplifying RNA vaccines for infectious diseases. 28:117–129. https://doi.org/10.1038/s41434-020-00204-y
Liu L, Wang P, Nair MS, Yu J, Rapp M, Wang Q, Luo Y, Chan JF-W, Sahi V, Figueroa A (2020) Potent neutralizing antibodies against multiple epitopes on SARS-CoV-2 spike. Nature 584:450–456. https://doi.org/10.1038/s41586-020-2571-7
Gamelin FX, Baquet G, Berthoin S, Thevenet D, Nourry C, Nottin S, Bosquet L (2021) Scientific rationale for developing potent RBD-based vaccines targeting COVID-19. npj Vaccines 6:128. https://doi.org/10.1038/s41541-021-00393-6
European Medicines Agency (EMA). (2021). Comirnaty EPAR public assessment report. CHMP Committee for Medicinal Products for Human Use. https://www.ema.europa.eu/en/documents/assessment-report/comirnaty-epar-public-assessment-report_en.pdf . Accessed 19 February 2021
Selmin F, Musazzi UM, Franzè S, Scarpa E, Rizzello L, Procacci P, Minghetti P (2021) Pre-drawn syringes of Comirnaty for an efficient COVID-19 mass vaccination: demonstration of stability. Pharmaceutics 13:1029. https://doi.org/10.3390/pharmaceutics13071029
Gruber MF (2020) Emergency use authorization (EUA) for an unapproved product review memorandum, in FDA.gov. https://www.fda.gov/media/144416/download . Accessed 20 November 2020
Dolgin E (2020) COVID-19 vaccines poised for launch, but impact on pandemic unclear. Nat Biotechnol. https://doi.org/10.1038/d41587-020-00022-y.Accessed25November2020
Bahl K, Senn JJ, Yuzhakov O, Bulychev A, Brito LA, Hassett KJ, Laska ME, Smith M, Almarsson Ö, Thompson J (2017) Preclinical and clinical demonstration of immunogenicity by mRNA vaccines against H10N8 and H7N9 influenza viruses. Mol Ther 25:1316–1327. https://doi.org/10.1016/j.ymthe.2017.03.035
Reuben RC, Adogo LY (2021) SARS-CoV-2 vaccines–induced thrombotic thrombocytopenia: should we consider immuno-hypersensitivity? Rev Saude Publica 55:70. https://doi.org/10.11606/s1518-8787.2021055003855
Winter E, Pizzol CD, Locatelli C, Crezkynski-Pasa TB (2016) Development and evaluation of lipid nanoparticles for drug delivery: study of toxicity in vitro and in vivo. J Nanosci Nanotechnol 16:1321–1330. https://doi.org/10.1166/jnn.2016.11667
Ng KK, Lovell JF, Zheng G (2011) Lipoprotein-inspired nanoparticles for cancer theranostics. Acc Chem Res 44:1105–1113. https://doi.org/10.1021/ar200017e
Sellaturay P, Nasser S, Islam S, Gurugama P, Ewan PW (2021) Polyethylene glycol (PEG) is a cause of anaphylaxis to the Pfizer/BioNTech mRNA COVID-19 vaccine. Clin Exp Allergy 51:861. https://doi.org/10.1111/cea.13874
Banerji A, Wickner PG, Saff R, Stone CA Jr, Robinson LB, Long AA, Wolfson AR, Williams P, Khan DA, Phillips E (2021) mRNA vaccines to prevent COVID-19 disease and reported allergic reactions: current evidence and suggested approach. J Allergy Clin Immunol Pract 9:1423–1437. https://doi.org/10.1016/j.jaip.2020.12.047
Ulbricht J, Jordan R, Luxenhofer R (2014) On the biodegradability of polyethylene glycol, polypeptoids, and poly (2-oxazoline)s. Biomaterials 35:4848–4861. https://doi.org/10.1016/j.biomaterials.2014.02.029
Peckham H, de Gruijter NM, Raine C, Radziszewska A, Ciurtin C, Wedderburn LR, Rosser EC, Webb K, Deakin CT (2020) Male sex identified by global COVID-19 meta-analysis as a risk factor for death and ICU admission. Nat Commun 11:6317. https://doi.org/10.1038/s41467-020-19741-6
Saifer MG, Williams LD, Sobczyk MA, Michaels SJ, Sherman MR (2014) Selectivity of binding of PEGs and PEG-like oligomers to anti-PEG antibodies induced by methoxypolyethylene glycol-proteins. Mol Immunol 57:236–246. https://doi.org/10.1016/j.molimm.2013.07.014
Yang Q, Lai SK (2015) Anti-PEG immunity: emergence, characteristics, and unaddressed questions. Wiley Interdiscip Rev Nanomed Nanobiotechnol 7:655–677. https://doi.org/10.1002/wnan.1339
Zhang P, Sun F, Liu S, Jiang S (2016) Anti-PEG antibodies in the clinic: current issues and beyond PEGylation. J Control Release 244:184–193. https://doi.org/10.1016/j.jconrel.2016.06.040
Zhou Z-H, Stone CA, Jakubovic B, Phillips EJ, Sussman G, Park J, Hoang U, Kirshner SL, Levin R, Kozlowski S (2021) Anti-PEG IgE in anaphylaxis associated with polyethylene glycol. J Allergy Clin Immunol Pract 9:1731-1733.e3. https://doi.org/10.1016/j.jaip.2020.11.011
Stone CA Jr, Liu Y, Relling MV, Krantz MS, Pratt AL, Abreo A, Hemler JA, Phillips EJ (2019) Immediate hypersensitivity to polyethylene glycols and polysorbates: more common than we have recognized. J Allergy Clin Immunol Pract 7:1533-1540.e8. https://doi.org/10.1016/j.jaip.2018.12.003
Hershfield MS, Ganson NJ, Kelly SJ, Scarlett EL, Jaggers DA, Sundy JS (2014) Induced and pre-existing anti-polyethylene glycol antibody in a trial of every 3-week dosing of pegloticase for refractory gout, including in organ transplant recipients. Arthritis Res Ther 16:R63. https://doi.org/10.1186/ar4500
Market (2017) Pegloticase: withdrawal of its European marketing authorisation is welcome. Prescrire Int 26:71. https://www.ncbi.nlm.nih.gov/pubmed/30730621 , https://www.ema.europa.eu/en/documents/public-statement/public-statement-krystexxa-withdrawal-marketing-authorisation-european-union_en.pdf
Vrieze J (2020) Suspicions grow that nanoparticles in Pfizer’s COVID-19 vaccine trigger rare allergic reactions. Scienceinsider. https://doi.org/10.1126/science.abg2359 , https://www.science.org/content/article/suspicions-grow-nanoparticles-pfizer-s-covid-19-vaccine-trigger-rare-allergic-reactions . Accessed 21 Dec 2020
Coors EA, Seybold H, Merk HF, Mahler V (2005) Polysorbate 80 in medical products and non-immunologic anaphylactoid reactions. Ann Allergy Asthma Immunol 95:593–599. https://doi.org/10.1016/S1081-1206(10)61024-1
Carpenter T, Konig J, Hochfelder J, Siegel S, Gans M (2022) Polyethylene glycol and polysorbate testing in 12 patients before or after coronavirus disease 2019 vaccine administration. Ann Allergy Asthma Immunol 128:99–101. https://doi.org/10.1016/j.anai.2021.10.009
Sadoff J, Gray G, Vandebosch A, Cárdenas V, Shukarev G, Grinsztejn B, Goepfert PA, Truyers C, Fennema H, Spiessens B (2021) Safety and efficacy of single-dose Ad26.COV2.S vaccine against COVID-19. N Engl J Med 384:2187–2201. https://doi.org/10.1056/NEJMoa2101544
Baden LR, El Sahly HM, Essink B, Kotloff K, Frey S, Novak R, Diemert D, Spector SA, Rouphael N, Creech CB (2021) Efficacy and safety of the mRNA-1273 SARS-CoV-2 vaccine. N Engl J Med 384:403–416. https://doi.org/10.1056/NEJMoa2035389
Polack FP, Thomas SJ, Kitchin N, Absalon J, Gurtman A, Lockhart S, Perez JL, Marc GP, Moreira ED, Zerbini C (2020) Safety and efficacy of the BNT162b2 mRNA COVID-19 vaccine. N Engl J Med. https://doi.org/10.1056/NEJMoa2034577
Schwede K, Simon JC, Treudler R (2019) A case of severe immediate type reaction to macrogol and polysorbate 60 after intravaginal drug application. Eur J Dermatol 29:329–331. https://doi.org/10.1684/ejd.2019.3541
Wenande E, Garvey L (2016) Immediate-type hypersensitivity to polyethylene glycols: a review. Clin Exp Allergy 46:907–922. https://doi.org/10.1111/cea.12760
Rajpurkar M, O’Brien SH, Haamid FW, Cooper DL, Gunawardena S, Chitlur M (2016) Heavy menstrual bleeding as a common presenting symptom of rare platelet disorders: illustrative case examples. J Pediatr Adolesc Gynecol 29:537–541. https://doi.org/10.1016/j.jpag.2016.02.002
Soltani Hekmat A, Javanmardi K (2021) Possible risk of thrombotic events following Oxford-AstraZeneca COVID-19 vaccination in women receiving estrogen. BioMed Res Int 2021:7702863. https://doi.org/10.1155/2021/7702863
Hunter PR (2021) Thrombosis after COVID-19 vaccination. BMJ 373:n958. https://doi.org/10.1136/bmj.n958
Alghamdi AN, Alotaibi MI, Alqahtani AS, Al Aboud D, Abdel-Moneim AS (2021) BNT162b2 and ChAdOx1 SARS-CoV-2 post-vaccination side-effects among Saudi vaccinees. Front Med 8:1796. https://doi.org/10.3389/fmed.2021.760047
GOV.UK (2022) Coronavirus vaccine - summary of yellow card reporting in vigilance, safety alerts and guidance. https://www.gov.uk/government/publications/coronavirus-covid-19-vaccine-adverse-reactions/coronavirus-vaccine-summary-of-yellow-card-reporting . Updated 8 March 2023
Rela M, Jothimani D, Vij M, Rajakumar A, Rammohan A (2021) Auto-immune hepatitis following COVID vaccination. J Autoimmun 123:102688. https://doi.org/10.1016/j.jaut.2021.102688
Patrizio A, Ferrari SM, Antonelli A, Fallahi P (2021) A case of Graves’ disease and type 1 diabetes mellitus following SARS-CoV-2 vaccination. J Autoimmun 125:102738. https://doi.org/10.1016/j.jaut.2021.102738
Zavala-Miranda MF, González-Ibarra SG, Pérez-Arias AA, Uribe-Uribe NO, Mejia-Vilet JM (2021) New-onset systemic lupus erythematosus beginning as class V lupus nephritis after COVID-19 vaccination. Kidney Int 100:1340–1341. https://doi.org/10.1016/j.kint.2021.09.009
Waheed S, Bayas A, Hindi F, Rizvi Z, Espinosa PS (2021) Neurological complications of COVID-19: Guillain-Barre syndrome following Pfizer COVID-19 vaccine. Cureus 13:e13426. https://doi.org/10.7759/cureus.13426
Abramson M, Yu SM-W, Campbell KN, Chung M, Salem F (2021) IgA nephropathy after SARS-CoV-2 vaccination. Kidney Med 3:860–863. https://doi.org/10.1016/j.xkme.2021.05.002
Jara LJ, Vera-Lastra O, Mahroum N, Pineda C, Shoenfeld Y (2022) Autoimmune post-COVID vaccine syndromes: does the spectrum of autoimmune/inflammatory syndrome expand? Clin Rheumatol 41:1603–1609. https://doi.org/10.1007/s10067-022-06149-4
Aldén M, Olofsson Falla F, Yang D, Barghouth M, Luan C, Rasmussen M, De Marinis Y (2022) Intracellular reverse transcription of Pfizer-BioNTech COVID-19 mRNA vaccine BNT162b2 in vitro in human liver cell line. Curr Issues Mol Biol 44:1115–1126. https://doi.org/10.3390/cimb44030073
Merchant HA (2022) Comment on Aldén et al. Intracellular reverse transcription of Pfizer-BioNTech COVID-19 mRNA vaccine BNT162b2 in vitro in human liver cell line. Curr Issues Mol Biol 44:1661–1663. https://doi.org/10.3390/cimb44040113
Rijkers GT, van Overveld FJ (2021) The “original antigenic sin” and its relevance for SARS-CoV-2 (COVID-19) vaccination. Clin Immunol Commun 1:13–16. https://doi.org/10.1016/j.clicom.2021.10.001
Hoepel W, Chen H-J, Geyer CE, Allahverdiyeva S, Manz XD, de Taeye SW, Aman J, Mes L, Steenhuis M, Griffith GR (2021) High titers and low fucosylation of early human anti-SARS-CoV-2 IgG promote inflammation by alveolar macrophages. Sci Transl Med 13:eabf8654. https://doi.org/10.1126/scitranslmed.abf8654
Kurdoğlu Z (2021) Do the COVID-19 vaccines cause menstrual irregularities? Int J Womens Health Reprod Sci 9:158–159. https://doi.org/10.15296/ijwhr.2021.29
Bozkurt B, Kamat I, Hotez PJ (2021) Myocarditis with COVID-19 mRNA vaccines. Circulation 144:471–484. https://doi.org/10.1161/CIRCULATIONAHA.121.056135
Seephetdee C, Bhukhai K, Buasri N, Leelukkanaveera P, Lerdwattanasombat P, Manopwisedjaroen S, Phueakphud N, Kuhaudomlarp S, Saphire EO, Thitithanyanont A (2022) Broad neutralization of SARS-CoV-2 variants by circular mRNA producing vFlip-X spike in mice. https://doi.org/10.1101/2022.03.17.484759
ClinicalTrials.gov (2021) SARS-CoV-2-Spike-Ferritin-Nanoparticle (SPFN) vaccine with ALFQ adjuvant for prevention of COVID-19 in healthy adults. (NCT04784767). https://clinicaltrials.gov/ct2/show/NCT04784767
Joh DY, Zimmers Z, Avlani M, Heggestad JT, Aydin HB, Ganson N, Kumar S, Fontes CM, Achar RK, Hershfield MS (2019) Architectural modification of conformal PEG-bottlebrush coatings minimizes anti-PEG antigenicity while preserving stealth properties. Adv Healthc Mater 8:1801177. https://doi.org/10.1002/adhm.201801177
Friedl JD, Nele V, De Rosa G, Bernkop-Schnürch A (2021) Bioinert, stealth, or interactive: how surface chemistry of nanocarriers determines their fate in vivo. Adv Funct Mater 31:2103347. https://doi.org/10.1002/adfm.202103347
Han X, Lu Y, Xie J, Zhang E, Zhu H, Du H, Wang K, Song B, Yang C, Shi Y (2020) Zwitterionic micelles efficiently deliver oral insulin without opening tight junctions. Nat Nanotechnol 15:605–614. https://doi.org/10.1038/s41565-020-0693-6
Xue W, Liu Y, Zhang N, Yao Y, Ma P, Wen H, Huang S, Luo Y, Fan H (2018) Effects of core size and peg coating layer of iron oxide nanoparticles on the distribution and metabolism in mice. Int J Nanomedicine 13:5719–5728. https://doi.org/10.2147/IJN.S165451
Yao X, Qi C, Sun C, Huo F, Jiang X (2023) Poly(ethylene glycol) alternatives in biomedical applications. Nano Today 48:101738. https://doi.org/10.1016/j.nantod.2022.101738
Patente TA, Pinho MP, Oliveira AA, Evangelista GC, Bergami-Santos PC, Barbuto JA (2019) Human dendritic cells: their heterogeneity and clinical application potential in cancer immunotherapy. Front Immunol 9:3176. https://doi.org/10.3389/fimmu.2018.03176
Tezera LB, Bielecka MK, Chancellor A, Reichmann MT, Shammari BA, Brace P, Batty A, Tocheva A, Jogai S, Marshall BG (2017) Dissection of the host-pathogen interaction in human tuberculosis using a bioengineered 3-dimensional model. Elife 6:e21283. https://doi.org/10.7554/eLife.21283
Geisbert TW, Pushko P, Anderson K, Smith J, Davis KJ, Jahrling PB (2002) Evaluation in nonhuman primates of vaccines against Ebola virus. Emerg Infect Dis 8:503–507. https://doi.org/10.3201/eid0805.010284
Vidal Oribe I, Venturini Díaz M, Hernández Alfonso P, del Pozo Gil M, González Mahave I, Lobera Labairu T (2022) Tolerance of SARS-CoV-2 vaccines with polyethylene glycol in allergic patients to polysorbate 80. J Investig Allergol Clin Immunol 32:403–405. https://doi.org/10.18176/jiaci.0772
Broyles AD, Banerji A, Barmettler S, Biggs CM, Blumenthal K, Brennan PJ, Breslow RG, Brockow K, Buchheit KM, Cahill KN (2020) Practical guidance for the evaluation and management of drug hypersensitivity: specific drugs. J Allergy Clin Immunol Pract 8:S16–S116. https://doi.org/10.1016/j.jaip.2020.08.002
Greenhawt M, Shaker M, Golden DB (2021) Peg/polysorbate skin testing has no utility in the assessment of suspected allergic reactions to SARS-CoV-2 vaccines. J Allergy Clin Immunol Pract 9:3321–3322. https://doi.org/10.1016/j.jaip.2021.06.025
Pitlick MM, Gonzalez-Estrada A, Park MA (2022) Graded COVID-19 vaccine administration: a safe alternative to vaccine avoidance. Ann Allergy Asthma Immunol 128:731–733. https://doi.org/10.1016/j.anai.2022.02.024
Liu X, Shaw RH, Stuart AS, Greenland M, Aley PK, Andrews NJ, Cameron JC, Charlton S, Clutterbuck EA, Collins AM (2021) Safety and immunogenicity of heterologous versus homologous prime-boost schedules with an adenoviral-vectored and mRNA COVID-19 vaccine (Com-COV): a single-blind, randomized, non-inferiority trial. Lancet 398:856–869. https://doi.org/10.1016/S0140-6736(21)01694-9
Yii A, Tay TR, Choo X, Koh M, Tee A, Wang DY (2018) Precision medicine in united airways disease: a “treatable traits” approach. Allergy 73:1964–1978. https://doi.org/10.1111/all.13496
Łoczechin A, Séron K, Barras A, Giovanelli E, Belouzard S, Chen Y-T, Metzler-Nolte N, Boukherroub R, Dubuisson J, Szunerits S (2019) Functional carbon quantum dots as medical countermeasures to human coronavirus. ACS Appl Mater Interfaces 11:42964–42974. https://doi.org/10.1021/acsami.9b15032
Huang X, Li M, Xu Y, Zhang J, Meng X, An X, Sun L, Guo L, Shan X, Ge J (2019) Novel gold nanorod-based HR1 peptide inhibitor for Middle East respiratory syndrome coronavirus. ACS Appl Mater Interfaces 11:19799–19807. https://doi.org/10.1021/acsami.9b04240
Neufurth M, Wang X, Tolba E, Lieberwirth I, Wang S, Schröder HC, Müller WE (2020) The inorganic polymer, polyphosphate, blocks binding of SARS-CoV-2 spike protein to ACE2 receptor at physiological concentrations. Biochem Pharmacol 182:114215. https://doi.org/10.1016/j.bcp.2020.114215
Tang H, Qin H, He S, Li Q, Xu H, Sun M, Li J, Lu S, Luo S, Mao P (2023) Anti-coronaviral nanocluster restrain infections of SARS-CoV-2 and associated mutants through virucidal inhibition and 3CL protease inactivation. Adv Sci 10:2207098. https://doi.org/10.1002/advs.202207098
Gattani V, Dawre S (2023) Development of favipiravir loaded PLGA nanoparticles entrapped in in-situ gel for treatment of COVID-19 via nasal route. J Drug Deliv Sci Technol 79:104082. https://doi.org/10.1016/j.jddst.2022.104082
Idris A, Davis A, Supramaniam A, Acharya D, Kelly G, Tayyar Y, West N, Zhang P, McMillan CL, Soemardy C (2021) A SARS-CoV-2 targeted siRNA-nanoparticle therapy for COVID-19. Mol Ther 29:2219–2226. https://doi.org/10.1016/j.ymthe.2021.05.004
Nadworny PL, Hickerson L, Holley-Harrison HD, Bloom DC, Grams TR, Edwards TG, Schultz GS, Burrell RE (2023) Treatment of infection and inflammation associated with COVID-19, multi-drug resistant pneumonia and fungal sinusitis by nebulizing a nanosilver solution. Nanomed Nanotechnol Biol Med 48:102654. https://doi.org/10.1016/j.nano.2023.102654
Saify Nabiabad H, Amini M, Demirdas S (2022) Specific delivering of RNAi using spike’s aptamer-functionalized lipid nanoparticles for targeting SARS-CoV-2: a strong anti-COVID drug in a clinical case study. Chem Biol Drug Des 99:233–246. https://doi.org/10.1111/cbdd.13978
Pokhrel LR, Williams F, Cook PP, O’Rourke D, Murray G, Akula SM (2022) Preclinical efficacy and safety of novel SNAT against SARS-CoV-2 using a hamster model. Drug Delivery Transl Res 12:3007–3016. https://doi.org/10.1007/s13346-022-01166-x
Zhou Y, Jiang X, Tong T, Fang L, Wu Y, Liang J, Xiao S (2020) High antiviral activity of mercaptoethane sulfonate functionalized Te/BSA nanostars against arterivirus and coronavirus. RSC Adv 10:14161–14169. https://doi.org/10.1039/D0RA01387K
Du T, Zhang J, Li C, Song T, Li P, Liu J, Du X, Wang S (2020) Gold/silver hybrid nanoparticles with enduring inhibition of coronavirus multiplication through multisite mechanisms. Bioconjug Chem 31:2553–2563. https://doi.org/10.1021/acs.bioconjchem.0c00506
Du T, Liang J, Dong N, Lu J, Fu Y, Fang L, Xiao S, Han H (2018) Glutathione-capped Ag2S nanoclusters inhibit coronavirus proliferation through blockage of viral RNA synthesis and budding. ACS Appl Mater Interfaces 10:4369–4378. https://doi.org/10.1021/acsami.7b13811
Hu C-MJ, Chang W-S, Fang Z-S, Chen Y-T, Wang W-L, Tsai H-H, Chueh L-L, Takano T, Hohdatsu T, Chen H-W (2017) Nanoparticulate vacuolar ATPase blocker exhibits potent host-targeted antiviral activity against feline coronavirus. Sci Rep 7:1343. https://doi.org/10.1038/s41598-017-13316-0
Jensen DMK, Cun D, Maltesen MJ, Frøkjaer S, Nielsen HM, Foged C (2010) Spray drying of siRNA-containing PLGA nanoparticles intended for inhalation. J Control Release 142:138–145. https://doi.org/10.1016/j.jconrel.2009.10.010
Lv X, Wang P, Bai R, Cong Y, Suo S, Ren X, Chen C (2014) Inhibitory effect of silver nanomaterials on transmissible virus-induced host cell infections. Biomaterials 35:4195–4203. https://doi.org/10.1016/j.biomaterials.2014.01.054
Valizadeh H, Abdolmohammadi-Vahid S, Danshina S, Gencer MZ, Ammari A, Sadeghi A, Roshangar L, Aslani S, Esmaeilzadeh A, Ghaebi M (2020) Nano-curcumin therapy, a promising method in modulating inflammatory cytokines in COVID-19 patients. Int Immunopharmacol 89:107088. https://doi.org/10.1016/j.intimp.2020.107088
Park HH, Park W, Lee YY, Kim H, Seo HS, Choi DW, Kwon HK, Na DH, Kim TH, Choy YB (2020) Bioinspired DNase-I-coated melanin-like nanospheres for modulation of infection-associated NETosis dysregulation. Adv Sci 7:2001940. https://doi.org/10.1002/advs.202001940
Lee YY, Park HH, Park W, Kim H, Jang JG, Hong KS, Lee J-Y, Seo HS, Na DH, Kim TH, Choy YB (2021) Long-acting nanoparticulate DNase-1 for effective suppression of SARS-CoV-2-mediated neutrophil activities and cytokine storm. Biomaterials 267:120389. https://doi.org/10.1016/j.biomaterials.2020.120389
Morad R, Akbari M, Rezaee P, Koochaki A, Maaza M, Jamshidi Z (2021) First principle simulation of coated hydroxychloroquine on Ag, Au, and Pt nanoparticles. Sci Rep 11:1–9. https://doi.org/10.1038/s41598-021-81617-6
Niemiec S, Hilton S, Wallbank A, Azeltine-Bannerman M, Louiselle A, Elajaili H, Allawzi A, Xu J, Mattson C, Dewberry L (2020) Cerium oxide nanoparticles conjugated to anti-inflammatory microRNA-146a prevent bleomycin-induced acute lung injury. Nanomed Nanotechnol Biol Med 34:102388. https://doi.org/10.1016/j.nano.2021.102388
Wang G, Gaikwad H, McCarthy M, Gonzalez-Juarrero M, Li Y, Armstrong M, Reisdorph N, Morrison T, Simberg D (2021) Lipid nanoparticle formulation of niclosamide (Nano NCM) effectively inhibits SARS-CoV-2 replication in vitro. Precis Nanomed 4:724–737. https://doi.org/10.33218/001c.18813
Zhang Q, Honko A, Zhou J, Gong H, Downs SN, Vasquez JH, Fang RH, Gao W, Griffiths A, Zhang L (2020) Cellular nanosponges inhibit SARS-CoV-2 infectivity. Nano Lett 20:5570–5574. https://doi.org/10.1021/acs.nanolett.0c02278
Starpharma (2020) SPL creates slow-release soluble DEP® remdesivir nanoparticle. https://starpharma.com/assets/asxannouncements/200901%20SPL%20creates%20slow%20release%20soluble%20DEP%20remdesivir%20nanoparticle.pdf
Khaitov M, Nikonova A, Shilovskiy I, Kozhikhova K, Kofiadi I, Vishnyakova L, Nikolskii A, Gattinger P, Kovchina V, Barvinskaia E (2021) Silencing of SARS-CoV-2 with modified siRNA-peptide dendrimer formulation. Allergy 76:2840–2854. https://doi.org/10.1111/all.14850
ClinicalTrials.gov (2021) Treatment of patients with mild coronavirus-19 (COVID-19) disease with methotrexate associated to LDL like nanoparticles (Nano-COVID19). (NCT04610567). https://clinicaltrials.gov/ct2/show/NCT04610567?term=nanoparticle&recrs=adeh&cond=COVID-19&draw=2&rank=5
ClinicalTrials.gov (2020) Efficacy and safety of MTX-loaded nanoparticles to treat severe COVID-19 patients. (NCT04352465). https://clinicaltrials.gov/ct2/show/study/NCT04352465?term=nanoparticle&recrs=abdeh&cond=COVID-19&draw=2&rank=1
ClinicalTrials.gov (2021) Colloidal silver, treatment of COVID-19. (NCT04978025). https://clinicaltrials.gov/ct2/show/NCT04978025?term=nanoparticle&recrs=abdeh&cond=COVID-19&draw=3&rank=19
Gambichler T, Hamdani N, Budde H, Sieme M, Skrygan M, Scholl L, Dickel H, Behle B, Ganjuur N, Scheel C (2021) Bullous pemphigoid after SARS-CoV-2 vaccination: spike protein-directed immunofluorescence confocal microscopy and T cell receptor studies. Br J Dermatol 186:1012–1013. https://doi.org/10.1111/bjd.20890
Cemşitoğlu N, Adışen E, Erdem Ö (2022) Lichen planus after CoronaVac: a rare complication of vaccines. J Eur Acad Dermatol Venereol 36:e202–e204. https://doi.org/10.1111/jdv.17906
Alghamdi FA, Khayyat ST, Alshareef MM, Wa F (2022) New-onset lichen planusiInduced by the Pfizer COVID-19 vaccine. Case reports in dermatological medicine New-onset cutaneous lichen planus triggered by COVID-19 vaccination. Case Rep Dermatol Med 2022:2082445. https://doi.org/10.1155/2022/2082445
Troeltzsch M, Berndt R (2021) Comment on “oral lichen planus following the administration of vector-based COVID-19 vaccine (Ad26. Cov2. S).” Authors’ reply Oral Dis 27:1327–1328. https://doi.org/10.1111/odi.14060
Kha C, Itkin A (2021) New-onset chilblains in close temporal association with mRNA-1273 (Moderna) vaccination. JAAD Case Reports 8:66–67. https://doi.org/10.1016/j.jdcr.2021.03.046
Toom S, Wolf B, Avula A, Peeke S, Becker K (2021) Familial thrombocytopenia flare-up following the first dose of mRNA-1273 COVID-19 vaccine. Am J Hematol 96:E107–E108. https://doi.org/10.1002/Fajh.26128
McMahon DE, Kovarik CL, Damsky W, Rosenbach M, Lipoff JB, Tyagi A, Chamberlin G, Fathy R, Nazarian RM, Desai SR (2022) Clinical and pathologic correlation of cutaneous COVID-19 vaccine reactions including V-REPP: a registry-based study. J Am Acad Dermatol 86:113–121. https://doi.org/10.1016/j.jaad.2021.09.002
Ackerman M, Henry D, Finon A, Binois R, Esteve E (2021) Persistent maculopapular rash after the first dose of Pfizer-BioNTech COVID-19 vaccine. J Eur Acad Dermatol Venereol 35:e430–e432. https://doi.org/10.1111/jdv.17248
Cyrenne B, Al-Mohammedi F, DeKoven J, Alhusayen R (2021) Pityriasis rosea-like eruptions following vaccination with BNT162b2 mRNA COVID-19 vaccine. J Eur Acad Dermatol Venereol 35:e485–e487. https://doi.org/10.1111/jdv.17342
Balingit A (2023) What is COVID arm? Healthline.com. https://www.healthline.com/health/adult-vaccines/covid-arm
Cirillo N (2021) Reported orofacial adverse effects of COVID-19 vaccines: the knowns and the unknowns. J Oral Pathol Med 50:424–427. https://doi.org/10.1111/jop.13165
Furer V, Zisman D, Kibari A, Rimar D, Paran Y, Elkayam O (2021) Herpes zoster following BNT162b2 mRNA COVID-19 vaccination in patients with autoimmune inflammatory rheumatic diseases: a case series. Rheumatology (Oxford) 60:e8–e9. https://doi.org/10.1093/rheumatology/keab345
Patlolla AK, Hackett D, Tchounwou PB (2015) Genotoxicity study of silver nanoparticles in bone marrow cells of Sprague-Dawley rats. Food Chem Toxicol 85:52–60. https://doi.org/10.1016/j.fct.2015.05.005
Yin N, Liu Q, Liu J, He B, Cui L, Li Z, Yun Z, Qu G, Liu S, Zhou Q (2013) Silver nanoparticle exposure attenuates the viability of rat cerebellum granule cells through apoptosis coupled to oxidative stress. Small 9:1831–1841. https://doi.org/10.1002/smll.201202732
Ziemińska E, Stafiej A, Strużyńska L (2014) The role of the glutamatergic NMDA receptor in nanosilver-evoked neurotoxicity in primary cultures of cerebellar granule cells. Toxicology 315:38–48. https://doi.org/10.1016/j.tox.2013.11.008
Sung JH, Ji JH, Park JD, Yoon JU, Kim DS, Jeon KS, Song MY, Jeong J, Han BS, Han JH (2009) Subchronic inhalation toxicity of silver nanoparticles. Toxicol Sci 108:452–461. https://doi.org/10.1186/1743-8977-7-20
Jia J, Li F, Zhou H, Bai Y, Liu S, Jiang Y, Jiang G, Yan B (2017) Oral exposure to silver nanoparticles or silver ions may aggravate fatty liver disease in overweight mice. Environ Sci Technol 51:9334–9343. https://doi.org/10.1021/acs.est.7b02752
Kim YS, Kim JS, Cho HS, Rha DS, Kim JM, Park JD, Choi BS, Lim R, Chang HK, Chung YH (2008) Twenty-eight-day oral toxicity, genotoxicity, and gender-related tissue distribution of silver nanoparticles in Sprague-Dawley rats. Inhal Toxicol 20:575–583. https://doi.org/10.1080/08958370701874663
Van der Zande M, Vandebriel RJ, Van Doren E, Kramer E, Herrera Rivera Z, Serrano-Rojero CS, Gremmer ER, Mast J, Peters RJ, Hollman PC (2012) Distribution, elimination, and toxicity of silver nanoparticles and silver ions in rats after 28-day oral exposure. ACS Nano 6:7427–7442. https://doi.org/10.1021/nn302649p
Trickler WJ, Lantz SM, Murdock RC, Schrand AM, Robinson BL, Newport GD, Schlager JJ, Oldenburg SJ, Paule MG, Slikker W Jr (2010) Silver nanoparticle-induced blood-brain barrier inflammation and increased permeability in primary rat brain microvessel endothelial cells. Toxicol Sci 118:160–170. https://doi.org/10.1093/toxsci/kfq244
Juling S, Böhmert L, Lichtenstein D, Oberemm A, Creutzenberg O, Thünemann AF, Braeuning A, Lampen A (2018) Comparative proteomic analysis of hepatic effects induced by nanosilver, silver ions, and nanoparticle coating in rats. Food Chem Toxicol 113:255–266. https://doi.org/10.1016/j.fct.2018.01.056
Zhurkov V, Savostikova O, Yurchenko V, Krivtsova E, Kovalenko M, Murav’eva L, Alekseeva A, Belyaeva N, Mikhailova R, Sycheva L (2017) Features of the mutagenic and cytotoxic effects of nanosilver and silver sulfate in mice. Nanotechnol 12:667–672. https://doi.org/10.1134/S1995078017060143
Pratsinis A, Hervella P, Leroux JC, Pratsinis SE, Sotiriou GA (2013) Toxicity of silver nanoparticles in macrophages. Small 9:2576–2584. https://doi.org/10.1002/smll.201202120
Pang S, Gao Y, Wang F, Wang Y, Cao M, Zhang W, Liang Y, Song M, Jiang G (2020) Toxicity of silver nanoparticles on wound healing: a case study of zebrafish fin regeneration model. Sci Total Environ 717:137178. https://doi.org/10.1016/j.scitotenv.2020.137178
Wen H, Dan M, Yang Y, Lyu J, Shao A, Cheng X, Chen L, Xu L (2017) Acute toxicity and genotoxicity of silver nanoparticle in rats. Plos One 12:e0185554. https://doi.org/10.1371/Fjournal.pone.0185554
Lu T, Qu Q, Lavoie M, Pan X, Peijnenburg W, Zhou Z, Pan X, Cai Z, Qian H (2020) Insights into the transcriptional responses of a microbial community to silver nanoparticles in a freshwater microcosm. Environ Pollut 258:113727. https://doi.org/10.1016/j.envpol.2019.113727
Sung JH, Ji JH, Yoon JU, Kim DS, Song Y, Jeong J, Han S, Han JH, Chung YH, Kim J (2008) Lung function changes in Sprague-Dawley rats after prolonged inhalation exposure to silver nanoparticles. Inhalation Toxicol 20:567–574. https://doi.org/10.1080/08958370701874671
Lee S-H, Hwang SH, Park JS, Park H-S, Shin YS (2016) Anaphylaxis to polyethylene glycol (Colyte®) in a patient with diverticulitis. J Korean Med Sci 31:1662–1663. https://doi.org/10.3346/jkms.2016.31.10.1662
Pidaparti M, Bostrom B (2012) Comparison of allergic reactions to Pegasparaginase given intravenously versus intramuscularly. Pediatr Pediatr Blood Cancer 59:436–439. https://doi.org/10.1002/pbc.23380
Moreno A, Pitoc GA, Ganson NJ, Layzer JM, Hershfield MS, Tarantal AF, Sullenger BA (2019) Anti-PEG antibodies inhibit the anticoagulant activity of pegylated aptamers. Cell Chem Biol 26:634-644.e3. https://doi.org/10.1016/j.chembiol.2019.02.001
Sung JH, Ji JH, Park JD, Song MY, Song KS, Ryu HR, Yoon JU, Jeon KS, Jeong J, Han BS (2011) Subchronic inhalation toxicity of gold nanoparticles. Part Fibre Toxicol 8:1–18. https://doi.org/10.1186/1743-8977-8-16
Kim K-T, Zaikova T, Hutchison JE, Tanguay RL (2013) Gold nanoparticles disrupt zebrafish eye development and pigmentation. Toxicol Sci 133:275–288. https://doi.org/10.1093/toxsci/kft081
Hext PM, Tomenson JA, Thompson P (2005) Titanium dioxide: Inhalation toxicology and epidemiology. Ann Occup Hyg 49:461–472. https://doi.org/10.1093/annhyg/mei012
Zhang L, Bai R, Li B, Ge C, Du J, Liu Y, Le Guyader L, Zhao Y, Wu Y, He S (2011) Rutile TiO 2 particles exert size and surface coating dependent retention and lesions on the murine brain. Toxicol Lett 207:73–81. https://doi.org/10.1016/j.toxlet.2011.08.001
Wang J, Li Y, Li W, Chen C, Li B, Zhao Y (2008) Biological effect of intranasally instilled titanium dioxide nanoparticles on female mice. NANO 3:279–285. https://doi.org/10.1142/S1793292008001325
Mohammadipour A, Fazel A, Haghir H, Motejaded F, Rafatpanah H, Zabihi H, Hosseini M, Bideskan AE (2014) Maternal exposure to titanium dioxide nanoparticles during pregnancy; impaired memory and decreased hippocampal cell proliferation in rat offspring. Environ Toxicol Pharmacol 37:617–625. https://doi.org/10.1016/j.etap.2014.01.014
Hong F, Ji L, Zhou Y, Wang L (2017) Retracted: pulmonary fibrosis of mice and its molecular mechanism following chronic inhaled exposure to TiO2 nanoparticles. Environ Toxicol 33:711. https://doi.org/10.1002/TOX.22493
Chen J, Dong X, Zhao J, Tang G (2009) In vivo acute toxicity of titanium dioxide nanoparticles to mice after intraperitoneal injection. J Appl Toxicol 29:330–337. https://doi.org/10.1002/jat.1414
Download references
Acknowledgements
The authors thank Dr. Jianfeng Jin and Prof. Jenneke Klein-Nulend for their outstanding help in editing the manuscript. Also, we thank David Alexander for his careful reading of the manuscript.
Author information
Authors and affiliations.
Department of Medical Biotechnology, School of Medicine, Shahid Sadoughi University of Medical Sciences, Yazd, Iran
Fatemeh Araste
Department of Oral Cell Biology, Academic Centre for Dentistry Amsterdam (ACTA), University of Amsterdam and Vrije Universiteit Amsterdam, Amsterdam Movement Sciences, Gustav Mahlerlaan, 3004, 1081 LA, Amsterdam, The Netherlands
Astrid Diana Bakker & Behrouz Zandieh-Doulabi
You can also search for this author in PubMed Google Scholar
Contributions
B. Zandieh-Doulabi had the idea for the article. F. Araste performed the literature search and data analysis. F. Araste and B. Zandieh-Doulabi drafted the work, and all authors critically revised it.
Corresponding author
Correspondence to Behrouz Zandieh-Doulabi .
Ethics declarations
Conflict of interest.
The authors declare no competing interests.
Additional information
Publisher's note.
Springer Nature remains neutral with regard to jurisdictional claims in published maps and institutional affiliations.
Rights and permissions
Open Access This article is licensed under a Creative Commons Attribution 4.0 International License, which permits use, sharing, adaptation, distribution and reproduction in any medium or format, as long as you give appropriate credit to the original author(s) and the source, provide a link to the Creative Commons licence, and indicate if changes were made. The images or other third party material in this article are included in the article's Creative Commons licence, unless indicated otherwise in a credit line to the material. If material is not included in the article's Creative Commons licence and your intended use is not permitted by statutory regulation or exceeds the permitted use, you will need to obtain permission directly from the copyright holder. To view a copy of this licence, visit http://creativecommons.org/licenses/by/4.0/ .
Reprints and permissions
About this article
Araste, F., Bakker, A.D. & Zandieh-Doulabi, B. Potential and risks of nanotechnology applications in COVID-19-related strategies for pandemic control. J Nanopart Res 25 , 229 (2023). https://doi.org/10.1007/s11051-023-05867-3
Download citation
Received : 09 December 2022
Accepted : 09 October 2023
Published : 09 November 2023
DOI : https://doi.org/10.1007/s11051-023-05867-3
Share this article
Anyone you share the following link with will be able to read this content:
Sorry, a shareable link is not currently available for this article.
Provided by the Springer Nature SharedIt content-sharing initiative
- Antiviral strategies
- Health effects
- Nanoparticles
- Nanoparticle-associated risks
- Nanotechnology
- Pandemic control
- Find a journal
- Publish with us
- Track your research

IMAGES
VIDEO
COMMENTS
Nanotechnology is a known field of research since last century. Since "nanotechnology" was presented by Nobel laureate Richard P. Feynman during his well famous 1959 lecture "There's Plenty of Room at the Bottom" (Feynman, 1960), there have been made various revolutionary developments in the field of nanotechnology.Nanotechnology produced materials of various types at nanoscale level.
2.2. Discovery of C, Ag, Zn, Cu, and Au nanoparticles. Carbon NPs were found in 1991, and Iijima and Ichihashi announced the single-wall carbon nanotube synthesis with a diameter of 1 nanometer in 1993 (Chen et al., 2021).Carbon nanotubes (CNTs), also known as Bucky tubes, are a kind of nanomaterial made up of a two-dimensional hexagonal lattice of carbon atoms.
Nanoparticles are particles that exist on a nanometre scale (i.e., below 100 nm in at least one dimension). They can possess physical properties such as uniformity, conductance or special optical ...
Journal of Nanoparticle Research is a peer-reviewed journal that delves into concepts, properties, phenomena, and processes of structures at the nanoscale. ... Research paper 05 April 2024 Article: 75 Layer-by-layer chitosan and gold nanoparticles as surface-enhanced Raman scattering substrate. Paulo Henrique de Melo Toledo ...
International Science Congress As sociation 1. A Review on Nanoparticles: Their Synthesis and Ty pes. Saba Hasan. Amity Institute of Biotechnology, Amity University Uttar Pradesh (Lucknow Campus ...
Microfluidic production of silver nanoparticles demonstrates ability for on demand synthesis of a wide size distribution of particles. Katelyn J. Langguth. Sara Maccagnano-Zachera. Joshua Heinemann. Research paper Open access 21 February 2024 Article: 40.
Nanoparticle chemistry is a relatively young branch of chemical research. Even 30 years ago, these words would have sounded puzzling to many scientists despite the fact that nanoparticles, primarily in the form of dust and smoke, have always existed in nature. Nanoparticles were utilized in construction materials, pigments, and stained glass ...
7.2.1. Chemical Reduction. Chemical reduction is an effective wet-chemical method for making zero-valent nanoparticles based on chemical-reducing aqueous salts of metals, such as silver nitrate (AgNO 3) in the case of synthesis of silver nanoparticles, for instance.To reduce the precursor metal salt, at least one reducing agent is used to produce electrons for metal ions that reduce them to ...
Silica nanoparticles (SNPs) have shown great applicability potential in a number of fields like chemical, biomedical, biotechnology, agriculture, environmental remediation and even wastewater purification. With remarkably instinctive properties like mesoporous structure, high surface area, tunable pore size/diameter, biocompatibility, modifiability and polymeric hybridizability, the SNPs are ...
In regenerative medicine, stem-cell-derived extracellular vesicles are emerging as cell-free nanotherapeutics. Here, the authors show that coating these nanovesicles with blood proteins such as ...
Nanoparticles. Nanoparticles (NPs) are technically defined as particles with one dimension less than 100 nm with unique properties usually not found in bulk samples of the same material [].Depending on the nanoparticle's overall shape, these can be classified as 0D, 1D, 2D or 3D [].The basic composition of nanoparticles is quite complex, comprising the surface layer, the shell layer, and the ...
Conjugated polymer nanoparticles are profoundly flexible nano-organized materials that can conceivably discover applications in different branches of science and engineering, for example, optoelectronics, photonics, bio-imaging, bio-detecting and nanomedicine. ... the recent developments in research in various area of polymers can empower ...
View a PDF of the paper titled Element-specific ultrafast lattice dynamics in FePt nanoparticles, by Diego Turenne and 16 other authors View PDF Abstract: Light-matter interaction at the nanoscale in magnetic alloys and heterostructures is a topic of intense research in view of potential applications in high-density magnetic recording.
NANOPARTICLES AND THEIR APPLICATIONS - A MINI REVIEW. M. Anto Godwin*, K. Mahitha Shri, M. Balaji. Department of Nan otechnology, Noorul Islam Centre for Higher Education, Noorul Islam U ...
The applications of copper (Cu) and Cu-based nanoparticles, which are based on the earth-abundant and inexpensive copper metal, have generated a great deal of interest in recent years, especially in the field of catalysis. The possible modification of the chemical and physical properties of these nanoparticles using different synthetic strategies and conditions and/or via postsynthetic ...
According to the findings of Van et al. 16, chitosan nanoparticles created by spray drying had a size distribution of 300 to 1500 nm, with an average size of 420 nm, between 500 and 2500 nm, with ...
Considering metal oxide nanoparticles as important technological materials, authors provide a comprehensive review of researches on metal oxide nanoparticles, their synthetic strategies, and techniques, nanoscale physicochemical properties, defining specific industrial applications in the various fields of applied nanotechnology. This work expansively reviews the recent developments of ...
1. INTRODUCTION. Nanotechnology is a relatively new branch of science that has found a wide range of applications that range from energy production to industrial production processes to biomedical applications. 1 One of the key applications is in biology and biomedical research. Nanoparticles (NPs) can be engineered to possess unique composition and functionalities, which can provide novel ...
Utilizing laser ablation for the generation of noble metal nanoparticles can be considered as a green technique, as there is no need for stabilizing agents or other chemicals. 51 A wide range of nanomaterials can be produced through this technique, such as metal nanoparticles, 52 carbon nanomaterials, 53,54 oxide composites, 55 and ceramics. 56 ...
Particles with size in the range of 1-100 nm, considered in any dimension, are called nanoparticles (NPs). Among various nanoparticles, nickel oxide nanoparticles (NNPs) are important because of their physical, chemical, and biological properties. In the past two decades, significant research has been done on the synthesis of NNPs.
Abstract. Current research into the role of engineered nanoparticles in drug delivery systems (DDSs) for medical purposes has developed numerous fascinating nanocarriers. This paper reviews the various conventionally used and current used carriage system to deliver drugs. Due to numerous drawbacks of conventional DDSs, nanocarriers have gained ...
Characterization of Se nanoparticles. In this study, a facile wet chemical method was utilized for synthesis of monodisperse SeNPs by Ascorbic acid with biocompatibility and good reducing properties.
Nanoparticles (NPs) of iron oxide are dispersed in mixtures of water and ionic liquid, here ethylammonium nitrate (EAN) and the interactions NP/NP and NP/solvent are studied. They are analysed by Small Angle X-Ray Scattering and Dynamic Light Scattering coupled to Forced Rayleigh Scattering from 22° C to 80° C. ... Paper. Submitted 19 Feb ...
Recent advances in nanoscience and nanotechnology radically changed the way we diagnose, treat, and prevent various diseases in all aspects of human life. Silver nanoparticles (AgNPs) are one of the most vital and fascinating nanomaterials among several metallic nanoparticles that are involved in biomedical applications. ... This paper was ...
Throughout the twentieth century, viral infections significantly impacted global health, causing millions of deaths worldwide. To combat these diseases, nanotechnology has emerged as a promising approach in the development of antiviral agents such as biosensors, nanoprobes, virus-like particles (VLPs), and functionalized nanoparticles [1,2,3].The COVID-19 pandemic highlighted the importance of ...