- Open access
- Published: 17 November 2011

Effects of abiotic stress on plants: a systems biology perspective
- Grant R Cramer 1 ,
- Kaoru Urano 2 ,
- Serge Delrot 3 ,
- Mario Pezzotti 4 &
- Kazuo Shinozaki 2
BMC Plant Biology volume 11 , Article number: 163 ( 2011 ) Cite this article
104k Accesses
740 Citations
6 Altmetric
Metrics details
The natural environment for plants is composed of a complex set of abiotic stresses and biotic stresses. Plant responses to these stresses are equally complex. Systems biology approaches facilitate a multi-targeted approach by allowing one to identify regulatory hubs in complex networks. Systems biology takes the molecular parts (transcripts, proteins and metabolites) of an organism and attempts to fit them into functional networks or models designed to describe and predict the dynamic activities of that organism in different environments. In this review, research progress in plant responses to abiotic stresses is summarized from the physiological level to the molecular level. New insights obtained from the integration of omics datasets are highlighted. Gaps in our knowledge are identified, providing additional focus areas for crop improvement research in the future.
Recent advances in biotechnology have dramatically changed our capabilities for gene discovery and functional genomics. For the first time, we can now obtain a holistic "snapshot" of a cell with transcript, protein and metabolite profiling. Such a "systems biology" approach allows for a deeper understanding of physiologically complex processes and cellular function [ 1 ]. New models can be formed from the plethora of data collected and lead to new hypotheses generated from those models.
Understanding the function of genes is a major challenge of the post-genomic era. While many of the functions of individual parts are unknown, their function can sometimes be inferred through association with other known parts, providing a better understanding of the biological system as a whole. High throughput omics technologies are facilitating the identification of new genes and gene function. In addition, network reconstructions at the genome-scale are key to quantifying and characterizing the genotype to phenotype relationships [ 2 ].
In this review, we summarize recent progress on systematic analyses of plant responses to abiotic stress to include transcriptomics, metabolomics, proteomics, and other integrated approaches. Due to space limitations, we try to emphasize important perspectives, especially from what systems biology and omics approaches have provided in recent research on environmental stresses.
Plant responses to the environment are complex
Plants are complex organisms. It is difficult to find an estimate of the total number of cells in a plant. Estimates of the number of cells in the adaxial epidermal layer and palisade mesophyll of a simple Arabidopsis leaf are approximately 27,000 and 57,000 cells, respectively [ 3 ]. Another estimate of the adaxial side of the epidermal layer of the 7 th leaf of Arabidopsis was close to 100,000 cells [ 4 ] per cm 2 of leaf area. An Arabidopsis plant can grow as large as 14 g fresh weight with a leaf area of 258 cm 2 (11 g fresh weight) [ 5 ]. Thus, we estimate that a single Arabidopsis plant could have approximately 100 million cells (range of 30 to 150 million cells assuming 2.4 to 11 million cells per g fresh weight). A one million Kg redwood tree could possibly have 70 trillion cells assuming a cell size 100 times larger than an Arabidopsis cell. Combine that with developmental changes, cell differentiation and interactions with the environment and it is easy to see that there are an infinite number of permutations to this complexity.
There is additional complexity within the cell with multiple organelles, interactions between nuclear, plastidial and mitochondrial genomes, and between cellular territories that behave like symplastically isolated domains that are able to exchange transcription factors controlling gene expression and developmental stages across the plasmodesmata. A typical plant cell has more than 30,000 genes and an unknown number of proteins, which can have more than 200 known post-translational modifications (PTMs). The molecular responses of cells (and plants) to their environment are extremely complex.
Environmental limits to crop production
In 1982, Boyer indicated that environmental factors may limit crop production by as much as 70% [ 6 ]. A 2007 FAO report stated that only 3.5% of the global land area is not affected by some environmental constraint (see Table three point seven in http://www.fao.org/docrep/010/a1075e/a1075e00.htm ). While it is difficult to get accurate estimates of the effects of abiotic stress on crop production (see different estimates in Table 1 ), it is evident that abiotic stress continues to have a significant impact on plants based upon the percentage of land area affected and the number of scientific publications directed at various abiotic stresses (Table 1 ). If anything the environmental impacts are even more significant today; yields of the "big 5" food crops are expected to decline in many areas in the future due to the continued reduction of arable land, reduction of water resources and increased global warming trends and climate change [ 7 ].
This growing concern is reflected in the increasing number of publications focused on abiotic stresses. For example, since the pivotal review of systems biology by Kitano in 2002 [ 1 ], the number of papers published on abiotic stress in plants using a systems biology approach has increased exponentially (Figure 1 ).
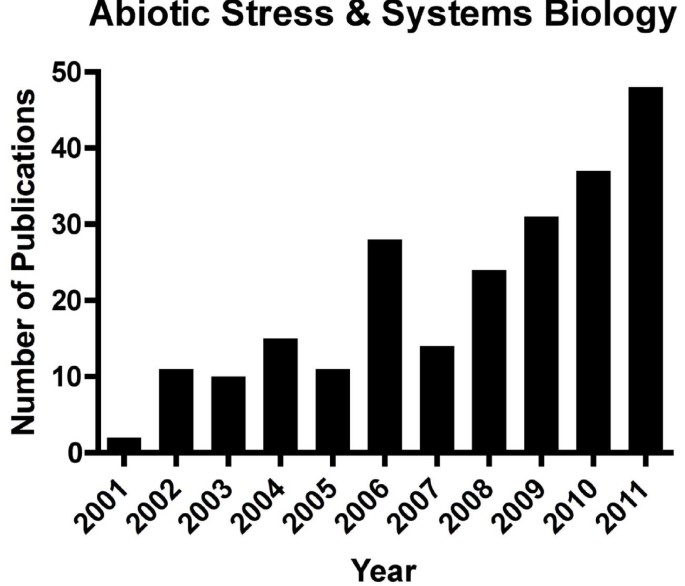
The number of publications per year related to systems biology and abiotic stress . Key words used in the search of PubMed included: plant, systems biology, and abiotic stress (including stress sub-terms; e.g. drought or water deficit or dehydration). *The number for the year 2011 was estimated by doubling the 6-month value.
Multiple factors limit plant growth
Fundamentally, plants require energy (light), water, carbon and mineral nutrients for growth. Abiotic stress is defined as environmental conditions that reduce growth and yield below optimum levels. Plant responses to abiotic stresses are dynamic and complex [ 8 , 9 ]; they are both elastic (reversible) and plastic (irreversible).
The plant responses to stress are dependent on the tissue or organ affected by the stress. For example, transcriptional responses to stress are tissue or cell specific in roots and are quite different depending on the stress involved [ 10 ]. In addition, the level and duration of stress (acute vs chronic) can have a significant effect on the complexity of the response [ 11 , 12 ].
Water deficit inhibits plant growth by reducing water uptake into the expanding cells, and alters enzymatically the rheological properties of the cell wall; for example, by the activity of ROS (reactive oxygen species) on cell wall enzymes [ 8 ]. In addition, water deficit alters the cell wall nonenzymatically; for example, by the interaction of pectate and calcium [ 13 ]. Furthermore, water conductance to the expanding cells is affected by aquaporin activity and xylem embolism [ 14 – 17 ]. The initial growth inhibition by water deficit occurs prior to any inhibition of photosynthesis or respiration [ 18 , 19 ].
The growth limitation is in part due to the fundamental nature of newly divided cells encasing the xylem in the growing zone [ 20 , 21 ]. These cells act as a resistance to water flow to the expanding cells in the epidermis making it necessary for the plant to develop a larger water potential gradient. Growth is limited by the plant's ability to osmotically adjust or conduct water. The epidermal cells can increase the water potential gradient by osmotic adjustment, which may be largely supplied by solutes from the phloem. Such solutes are supplied by photosynthesis that is also supplying energy for growth and other metabolic functions in the plant. With long-term stress, photosynthesis declines due to stomatal limitations for CO 2 uptake and increased photoinhibition from difficulties in dissipating excess light energy [ 12 ].
One of the earliest metabolic responses to abiotic stresses and the inhibition of growth is the inhibition of protein synthesis [ 22 – 25 ] and an increase in protein folding and processing [ 26 ]. Energy metabolism is affected as the stress becomes more severe (e.g. sugars, lipids and photosynthesis) [ 12 , 27 , 28 ]. Thus, there are gradual and complex changes in metabolism in response to stress.
Central regulators limit key plant processes
The plant molecular responses to abiotic stresses involve interactions and crosstalk with many molecular pathways [ 29 ]. Systems biology and omics approaches have been used to elucidate some of the key regulatory pathways in plant responses to abiotic stress.
One of the earliest signals in many abiotic stresses involve ROS and reactive nitrogen species (RNS), which modify enzyme activity and gene regulation [ 30 – 32 ]. ROS signaling in response to abiotic stresses and its interactions with hormones has been thoroughly reviewed [ 32 ]. ROS and RNS form a coordinated network that regulates many plant responses to the environment; there are a large number of studies on the oxidative effects of ROS on plant responses to abiotic stress, but only a few studies documenting the nitrosative effects of RNS [ 30 ].
Hormones are also important regulators of plant responses to abiotic stress (Figure 2 ). The two most important are abscisic acid (ABA) and ethylene [ 33 ]. ABA is a central regulator of many plant responses to environmental stresses, particularly osmotic stresses [ 9 , 34 – 36 ]. Its signaling can be very fast without involving transcriptional activity; a good example is the control of stomatal aperture by ABA through the biochemical regulation of ion and water transport processes [ 35 ]. There are slower responses to ABA involving transcriptional responses that regulate growth, germination and protective mechanisms.

A simplified working model of a signaling network of plant responses to abiotic stress . Ovals represent proteins, metabolites or processes. Metabolites have magenta color. Phosphorylated proteins have red circles with a P inside. Sumoylated protein has an orange circle with an S inside. The solid purple circle indicates that DREB2 needs modification to be activated. Solid lines represent direct connections; dotted lines represent indirect connections (acting through some intermediate molecule). The gray line indicates that this reaction has not been shown in plants. Not all linkages and details of stress and hormone effects are shown in this diagram in order to simplify the model. Abbreviations: ABA (abscisic acid), ANAC (Arabidopsis NAC domain-containing protein), CAMTA (calmodulin-binding transcription activator), CBL (calcineurin B-like interacting protein kinase), CCA (circadian clock associated), CPK (calcium-dependent protein kinase), DREB/CBF (dehydration response element binding protein/C-repeat binding factor), ETR1 (ethylene response 1), GCN2 (general control non-repressible 2), HSF (heat shock factor), ICE (inducer of CBF expression), MAPK (mitogen-activated protein kinase), LHY (late elongated hypocotyl), PA (phosphatidic acid), PP2C (protein phosphatase 2C), PRR (pseudo response regulator), PYR/PYL/RCAR (ABA receptors), RNS (reactive nitrogen species), ROS (reactive oxygen species), SIZ (SAP and Miz domain protein), SnRK (sucrose nonfermenting-1 related kinase), TFs (transcription factors), TOR (target of rapamycin), ZAT (zinc finger protein).
Recently, the essential components of ABA signaling have been identified, and their mode of action was clarified [ 37 ]. The current model of ABA signaling includes three core components, receptors (PYR/PYL/RCAR), protein phosphatases (PP2C) and protein kinases (SnRK2/OST1) [ 38 , 39 ]. The PYR/PYL/RCAR proteins were identified as soluble ABA receptors by two independent groups [ 38 , 39 ]. The 2C-type protein phosphatases (PP2C) including ABI1 and ABI2, were first identified from the ABA-insensitive Arabidopsis mutants abi1-1 and abi2-1 , and they act as global negative regulators of ABA signaling [ 40 ]. SNF1-related protein kinase 2 (SnRK2) is a family of protein kinases isolated as ABA-activated protein kinases [ 41 , 42 ]. In Arabidopsis, three members of this family, SRK2D/SnRK2.2, SRK2E/OST1/SnRK2.6, and SRK2I/SnRK2.3, regulate ABA signaling positively and globally, as shown in the triple knockout mutant srk2d srk2e srk2i ( srk2dei )/ snrk2.2 snrk2.3 snrk2.6 , which lacks ABA responses [ 43 ]. The PYR/PYL/RCAR - PP2C - SnRK2 complex plays a key role in ABA perception and signaling.
Studies of the transcriptional regulation of dehydration and salinity stresses have revealed both ABA-dependent and ABA-independent pathways [ 44 ]. Cellular dehydration under water limited conditions induces an increase in endogenous ABA levels that trigger downstream target genes encoding signaling factors, transcription factors, metabolic enzymes, and others [ 44 ]. In the vegetative stage, expression of ABA-responsive genes is mainly regulated by bZIP transcription factors (TFs) known as AREB/ABFs, which act in an ABA-responsive-element (ABRE) dependent manner [ 45 – 47 ]. Activation of ABA signaling cascades result in enhanced plant tolerance to dehydration stress. In contrast, a dehydration-responsive cis-acting element, DRE/CRT sequence and its DNA binding ERF/AP2-type TFs, DREB1/CBF and DREB2A, are related to the ABA-independent dehydration and temperature responsive pathways [ 44 ]. DREB1/CBFs function in cold-responsive gene expression [ 48 , 49 ], whereas DREB2s are involved in dehydration-responsive and heat-responsive gene expression [ 50 ].
Ethylene is also involved in many stress responses [ 51 – 53 ], including drought, ozone, flooding (hypoxia and anoxia), heat, chilling, wounding and UV-B light [ 31 , 33 , 53 ]. Ethylene signaling is well defined [ 51 , 52 ], and will not be discussed in detail here. There are known interactions between ethylene and ABA during drought [ 31 ], fruit ripening [ 54 , 55 ], and bud dormancy [ 56 ]. All of these interactions make the plant response to stress very complex [ 12 , 31 , 52 ].
In yeast, the well-documented central regulators of protein synthesis and energy are SnRK1 (Snf1/AMPK), TOR1 and GCN2 [ 57 – 60 ]. These proteins are largely controlled by the phosphorylation of enzymes; all three are protein kinases acting as key hubs in the coordination of metabolism during stressful conditions [ 61 ]. In plants, TOR activity is inhibited by osmotic stress and ABA [ 62 ] and GCN2 activity is stimulated by UV-light, amino acid starvation, ethylene, and cold stress [ 63 ]. SnRK1 responds to energy depletion, such as low light, nutrient deprivation or hypoxic conditions [ 64 , 65 ], and interacts with both glucose and ABA signaling pathways [ 66 ]. One of the results of this coordinated response is the inhibition of protein synthesis.
Many abiotic stresses directly or indirectly affect the synthesis, concentration, metabolism, transport and storage of sugars. Soluble sugars act as potential signals interacting with light, nitrogen and abiotic stress [ 67 – 69 ] to regulate plant growth and development; at least 10% of Arabidopsis genes are sugar-responsive [ 68 ]. Mutant analysis has revealed that sugar signaling interacts with ethylene [ 70 ], ABA [ 71 , 72 ], cytokinins [ 73 ], and light [ 74 , 75 ]. In grapevine, sugar and ABA signaling pathways interact to control sugar transport. An ASR (ABA, stress-, and ripening-induced) protein isolated from grape berries is upregulated synergistically by ABA and sugars, and upregulates the expression of a hexose transporter [ 76 ]. VVSK1, a GSK3 type protein kinase, is also induced by sugars and ABA, and upregulates the expression of several hexose transporters [ 77 ].
Stresses such as sugar starvation and lack of light stimulate SnRK1 activity ([ 64 ]. Suc-P synthase (SPS), 3-hydroxy-3-methylglutaryl-CoA reductase, nitrate reductase, and trehalose-6-P synthase are negatively regulated by SnRK1 phosphorylation [ 78 ], indicating that SnRK1 modulates metabolism by phosphorylating key metabolic enzymes. Post-translational redox modulation of ADPG-pyrophosphorylase, a key control of starch synthesis, by SnRK1 provides an interesting example of interactions between phosphorylation, redox control and sugar metabolism [ 79 ]. In Arabidopsis, SnRK1 kinase activity is itself increased by GRIK1 and GRIK2, which phosphorylate a threonine residue of the SnRK1 catalytic subunit [ 78 ]. SnRK2 interacts with ABA for the control of stomatal aperture and participates in the regulation of plant primary metabolism. Constitutive expression of SnRK2.6 drastically boosts sucrose and total soluble sugar levels in leaves, presumably by controlling SPS expression [ 80 ].
Systems biology approach to abiotic stress
In the post-genomic era, comprehensive analyses using three systematic approaches or omics have increased our understanding of the complex molecular regulatory networks associated with stress adaptation and tolerance. The first one is 'transcriptomics' for the analysis of coding and noncoding RNAs, and their expression profiles. The second one is 'metabolomics' that is a powerful tool to analyze a large number of metabolites. The third one is 'proteomics' in which protein and protein modification profiles offer an unprecedented understanding of regulatory networks. Protein complexes involved in signaling have been analyzed by a proteomics approach [ 81 , 82 ]. Integration of the different omics analyses facilitates abiotic stress signaling studies allowing for more robust identifications of molecular targets for future biotechnological applications in crops and trees.
Co-expression analyses identify regulatory hubs
An important application of transcriptomics data is co-expression analysis of target genes using on-line analytical tools, such as ATTED-II (reviewed by [ 83 ]). This approach is very promising for understanding gene-gene correlations and finding master genes in target conditions.
In a series of pioneering papers, Hirai et al. [ 84 , 85 ] identified MYB transcription factors regulating glucosinolate biosynthesis in Arabidopsis in response to S and N deficiency using an integrated transcriptomics and metabolomics approach. Genes and metabolites in glucosinolate metabolism were found to be coordinately regulated [ 84 ]. Co-expression analysis was used to identify two MYB transcription factors that positively regulate glucosinolate metabolism [ 85 ]. Then a knock out mutant and ectopic expression of one of the transcription factors was used to validate its positive role in glucosinolate metabolism. Previously unidentified genes were assigned to this biosynthetic pathway and a regulatory network model was constructed [ 85 ].
Mao et al. [ 86 ] performed a gene co-expression network analysis of 1094 microarrays of Arabidopsis using a non-targeted approach. They identified 382 modules in this network. The top three modules with the most nodes were: photosynthesis, response to oxidative stress and protein synthesis. Many of the modules also involved responses to environmental stresses. They constructed a cold-induced gene network from a subset of microarrays. The response to auxin stimulus was the most over-represented of the 18 significant modules.
Carrera et al. [ 87 ] used the InferGene application to construct a regulatory model of the Arabidopsis genome. They used datasets from 1,486 microarray experiments. Ten genes were predicted to be the most central regulatory hubs influencing the largest number of genes. Included in this set were transcription factor genes involved in auxin (KAN3), gibberellin (MYB29), abscisic acid (MYB121), ethylene (ERF1), and stress responses (ANAC036). They computed the top 12 gene subnetworks; four of these were related to biotic and abiotic stresses. Eighty-five percent of the predicted interactions of the 25% most connected transcription factors were validated in AtRegNet, the Arabidopsis thaliana Regulator Network http://arabidopsis.med.ohio-state.edu/moreNetwork.html .
Lorenz et al [ 88 ] investigated the drought response of loblolly pine roots and identified a number of hubs in the transcriptional network. Highly ranked hubs included thioredoxin, an inositol transporter, cardiolipin synthase/phosphatidyl transferase, 9-cis-expoxycarotenoid dioxygenase, zeatin O-glucosyltransferase and a SnRK2 kinase. These genes are involved in phospholipid metabolism, ABA biosynthesis and signaling, and cytokinin metabolism; they appear to be important in stress mediation.
Weston et al [ 89 ] used weighted co-expression analysis to define six modules for Arabidopsis responses to abiotic stress. Two hubs in the common response module were an ankyrin-repeat protein and genes involved in Ca signaling. They created a compendium of genomic signatures and linked them to their co-expression analysis. Using the same approach, they extended their analyses to the responses of three different plant species to heat and light [ 90 ]. Species-specific responses were found involving heat tolerance, heat-shock proteins, ROS, oligosaccharide metabolism and photosynthesis.
Time-series analyses reveal multiple phases in stress responses
Time-series analyses allow one to distinguish between primary and secondary responses to stress. In a comprehensive time-series transcriptomics analysis of 7 abiotic stresses on different Arabidopsis organs [ 28 ], a core set of genes (50% were transcription factors) of non-specific responses for all stresses were elucidated. Included in this set were the AZF2, ZAT10 and ZAT12 transcription factors. This initial response is thought to be involved in the readjustment of energy homeostasis in response to the stress. With time (after 1 h) more stress-specific profiles developed.
Sun et al [ 91 ] applied a complexity metric to a set of time series data of Arabidopsis with 9 different abiotic stresses. They found that genes with a higher complexity metric had longer 5' intergenic regions and a greater density of cis-regulatory motifs than the genes with a low complexity metric. Many of the cis-regulatory motifs identified were associated with previously characterized stress responses.
Vanderauwera et al. [ 92 ] investigated the effects of hydrogen peroxide (H 2 O 2 ) signaling during high light stress using microarray analyses. They found that H 2 O 2 was not only heavily involved in signaling in high light stress, but also salinity, water deficit, heat and cold stress. H 2 O 2 was a key regulator of small and 70 kD heat shock proteins and many genes of the anthocyanin metabolic pathway. Anthocyanins appear to play an important role as antioxidants in plants. A specific UDP-glycosyltransferase (UGT74E2) was highly regulated by H 2 O 2 . In a subsequent study [ 93 ], UGT74E2 responded quickly to H 2 O 2 and glycosylated indole-3-butyric acid (IBA) modifying auxin homeostasis, plant morphology and improving stress tolerance to salinity and water deficit. Furthermore, auxin was found to interact with ABA, increasing the ABA sensitivity of the plant. Silencing a poly(ADP-ribose) polymerase improved high light stress tolerance in Arabidopsis [ 94 , 95 ]. Part of the improved abiotic stress tolerance was ascribed to improved energy-use efficiency and reduced oxidative stress [ 94 , 95 ].
Kusano et al. [ 96 ] conducted a time-series experiment on the effects of UV-B light on Arabidopsis using both metabolomics and transcriptomics analyses. They found that plants responded in two phases with an upregulation of primary metabolites in the first phase and the induction of protective secondary metabolites, especially phenolics, in the second phase. The induction of phenolics corresponded to transcripts involved in the phenylpropanoid pathway, but the transcripts for primary metabolism were less consistent indicating that this pathway may be regulated by other mechanisms (e.g. kinases).
The transcriptomic response to drought can vary with the time of day [ 97 ]. These responses seem to interact with hormonal and other stress pathways that naturally vary during the course of the day. A smaller set of core genes were identified that responded at all times of the day. This set was compared to two previous studies and was whittled down to just 19 genes, including a NF-YB transcription factor, several PP2Cs, a CIPK7, and a sulfate transporter.
Drought stress studies and microarray analyses of three different genotypes of poplar clones grown in two different locations revealed epigenetic regulation to the environment [ 98 ]. The tree clones that had a longer history in the environment showed greater changes in DNA methylation, thereby influencing their response to drought.
Shoot tip growth of grapevines was found to be much more sensitive to osmotic stress than gene expression in a time-series experiment of the effects of gradual osmotic stress on grapevine [ 27 ]. Proteomics data indicated that changes in protein expression preceded and were not well correlated with gene expression (G.R. Cramer, unpublished results). The integration of transcriptomics data and metabolomics data indicated distinct differences of the responses of salinity and an isosmotic water deficit [ 27 ]. Drought-stressed plants induced greater responses in processes needed for osmotic adjustment and protection against ROS and photoinhibition. Salinity induced greater responses in processes involved in energy metabolism, ion transport, protein synthesis and protein fate. A comparison to similar short-term stresses [ 11 ] indicated that a gradual, chronic stress response was more complex than an acute stress response.
The effect of water-deficit on Cabernet Sauvignon berries (a red wine grape) in the field was studied using transcriptomics, proteomics and metabolomics [ 99 – 102 ]. Integrated analyses confirmed that the phenylpropanoid pathway (including anthocyanin and stilbene biosynthesis) was upregulated by water deficit in a tissue-specific manner in the skins of the berries. Other metabolic pathways in the berries were affected by water deficit including ABA, amino acid, carotenoid, lipid, sugar and acid metabolism. Most of these changes were associated with improved quality characteristics of the fruit.
Likewise, Zamboni et al. [ 103 ] investigated berry development and withering in grapevine at the transcriptomics, proteomics and metabolomics levels. A multistep hypothesis-free approach from four developmental stages and three withering intervals, with integration achieved using a hierarchical clustering strategy (multivariate O2PLS technique), identified stage-specific functional networks of linked transcripts, proteins and metabolites, providing important insights into the key molecular processes that determine wine quality. A hypothesis-driven approach identified transcript, protein and metabolite variables involved in the molecular events underpinning withering, which predominantly reflected a general stress response. Berry ripening and withering are characterized by the accumulation of secondary metabolites such as acylated anthocyanins, but withering also involves the activation of osmotic and oxidative stress response genes and the production of stilbenes and taxifolin.
Usadel et al. [ 104 ] investigated the effects of cold temperatures over time using transcriptomics, metabolomics and enzyme activities. They found some enzyme activities and metabolites changed rapidly, whereas others changed more slowly. The early changes (6 h) in enzyme activities were poorly correlated with transcript abundance, but after 78 h these correlations were greatly improved. Much of the long-term changes in metabolism could be ascribed to the CBF regulon.
Caldana et al. [ 105 ] conducted a complex time-series experiment (22 time points) with differing temperatures and light intensities using both metabolomics and transcriptomics analyses. This high-resolution time series experiment revealed that metabolic activities respond more quickly than transcriptional activities, indicating a disconnect between metabolism and transcription in the early phases of stress response and indicating that enzymatic activities may play a significant role. There were common metabolic responses to the changing environment within 1 h of the change including a decrease in energy metabolism and translation and an increase in the transcription of genes involved in signaling cascades. At later time points, condition-dependent metabolism was revealed. For example, protein degradation and energy metabolism derived from amino acids occurred in warm temperatures and darkness. Amino acid catabolism appears to fuel the TCA cycle in the absence of photosynthesis.
Yun et al. [ 106 ] characterized the response of rice to a mild chilling stress (10°C). They found that transcriptional regulation consisted of three dynamic and complex phases over 96 h. The early transcriptional phase appeared to be triggered by oxidative signals (H 2 O 2 ) and lead to the subsequent induction of cellular defense and rescue mechanisms. Combining temporal co-expression data from microarrays with promoter motif enrichment analyses and oxidative responses, transcriptional regulatory network models for the different response phases were constructed. A bZIP-TGA transcription factor module (as1/ocs/TGA), one of seven transcription factor modules, was the most connected regulatory module in phase one. Each of the transcription factor modules consisted of clusters of transcription factors exhibiting combinatorial control of the chilling regulon. The speed of the response of this network was associated with chilling tolerance. Chilling-resistant genotypes had a much more rapid and pronounced response of this transcriptional regulatory network than chilling-sensitive genotypes. In addition, the transcription factors identified in this study were located within known growth and stress QTLs in the rice genome.
Integration of omics analysis identifies molecular networks functioning in abiotic stress responses
Integrated omics analyses have markedly increased our understanding of plant responses to various stresses. These analyses are important for comprehensive analyses of abiotic stress responses, especially the final steps of stress signal transduction pathways.
Integrated analyses of the transcriptome and the metabolome successfully demonstrate connections between genes and metabolites, elucidating a wide range of signal output from ABA under dehydration [ 107 ] and the DREB1/CBF transcription factors in response to low temperature [ 108 , 109 ]. Metabolite profiling reveals that ABA accumulates during dehydration, regulating the accumulation of various amino acids and sugars such as glucose and fructose. In particular, the dehydration-inducible accumulation of BCAAs (branch-chain amino acids), saccharopine, proline, and agmatine are correlated with the dehydration-inducible expression of their key biosynthetic genes ( BCAT2 , LKR/SDH , P5CS1 , and ADC2 , respectively), which are regulated by endogenous ABA [ 107 ]. In addition, metabolome analysis of transgenic Arabidopsis overexpressing DREB1A/CBF3 reveals that there is a striking similarity between the low-temperature regulated metabolome (monosaccharides, disaccharides, oligosaccharides and sugar alcohols) and that regulated by the DREB1A/CBF3 transcription factor [ 108 , 109 ]. In particular, the low-temperature-inducible accumulation of galactinol and raffinose is correlated with the expression of the Gols3 gene, which is a direct target of DREB1A/CBF3 [ 108 , 109 ]. Maruyama et al. [ 109 ] also analyzed DREB2A overexpression, which did not increase the level of any low-temperature regulated metabolites in transgenic plants. Overexpression of DREB2A-CA in transgenic plants increased their tolerance to dehydration stress, but only slightly increased their tolerance to freezing stress [ 50 ]. These results indicate that the increased tolerance to freezing stress in transgenic plants overexpressing DREB1A may depend on the accumulation of low-temperature regulated metabolites, especially sucrose, raffinose, galactinol, and myo -inositol. Similarly, transcriptomics and metabolomics analyses of PSEUDO RESPONSE REGULATOR (PRR) arrhythmic triple mutant revealed that the DREB1A/CBF gene and raffinose amounts appear to be regulated by the circadian clock, varying between day and night as if in anticipation of the colder night temperatures [ 110 ].
Comparing metabolomics between dehydration, salinity, light, heat or low temperature stress have identified metabolites that are generally important in abiotic stress responses or are specific to each stress [ 27 , 95 , 105 , 111 , 112 ]. In a metabolite profiling study of Arabidopsis responses to combined dehydration and heat stresses [ 95 ], heat stress reduced the toxicity of proline, indicating that during the more severe combined stress treatment, sucrose replaces proline in plants as the major osmoprotectant. Comparative metabolite analysis between Arabidopsis responding to heat shock and cold shock revealed that the majority of metabolites in response to heat shock overlapped with those produced in response to cold shock [ 109 , 113 ]. These results indicate that a metabolic network of compatible solutes includes proline, monosaccharides (glucose and fructose), galactinol, and raffinose, which have an important role in tolerance to temperature stress. Wienkoop et al. [ 112 ] identified a RNA-binding protein (ATGRP7) that increased in response to low temperature stress and decreased in response to high temperature stress. Its abundance was significantly correlated with glutamine and proline concentrations. While raffinose and galactinol concentrations were significant markers for temperature responses, their response was independent of the responses of ATGRP7, proline and glutamine.
Transcriptomics, metabolomics and enzyme activities were integrated in a comprehensive study of K deficiency [ 114 ]. Carbon and nitrogen metabolism were significantly affected by K deficiency. This integrated approach pinpointed that pyruvate kinase activity (not transcription) was inhibited directly by K deficiency and was primarily responsible for the metabolic disorders observed.
Systematic application of omics technologies has contributed to the development of stress-tolerant crops in the field
Many genes affect stress tolerance, but few of the identified genes have proven useful in the field. Due to the complexity of stress interactions and stress responses, relevant phenotyping needs to be performed (including field experiments) in abiotic stress studies if we are to make significant progress [ 113 ]. The following studies are discussed to highlight good examples of systems biology and omics approaches that have been used to identify key genes regulating stress tolerance and then followed with validation of those responses and phenotypes in multiple experiments including field conditions.
A SNAC1 gene was identified from microarray experiments of stress treatments on rice [ 115 ]. SNAC1 is a NAC transcription factor that induces the expression of a number of stress-tolerance genes and improves the drought and salt tolerance of rice in the field. The transgenic plants exhibited increased sensitivity to ABA and reduced water loss. In another drought stress study, a LEA (late embryogenesis abundant) gene was identified from microarray experiments of rice and was transformed and tested in the field under drought conditions through the T3 generation [ 116 ]. Spikelet fertility appears to be the main factor contributing to improved yields under drought conditions.
An exhaustive screen of greater than 1500 transcription factors in Arabidopsis identified approximately 40 transcription factors that when overexpressed, improved stress tolerance [ 117 ]. One of these transcription factors NF-YB1 was further characterized and shown to display significant drought tolerance in Arabidopsis. Microarray data of this overexpressing line showed few differences in gene expression and the genes identified were not known previously to be involved in drought tolerance. This functional genomics approach provided a new strategy for improving drought tolerance in plants. A homolog of NF-YB1 was cloned in maize (ZmNF-YB2), overexpressed and tested for drought tolerance in the greenhouse and field plots. The transgenic maize lines were more drought tolerant having increased chlorophyll content, photosynthesis, stomatal conductance and grain yields. One line consistently had more than 50% yield improvement in drought conditions over two different years.
Oh et al. [ 118 ] used microarrays to identify 42 AP2 transcription factors whose expressions were affected by stress. Two of these transcription factors, AP37 and AP59 were functionally characterized. The two transcription factors are closely related but have distinct differences in affecting rice phenotype. AP37 responded to drought, salinity, cold and ABA; over-expression improved stress tolerance to all three environmental conditions. AP59 responded to drought and salinity, but not cold or ABA, and improved stress tolerance to drought and salinity only. Both overexpressing lines showed improved photosynthetic efficiency under stress conditions. Overexpression of the transcription factors induced common and distinct sets of genes. T5 homozygous overexpressing lines of AP59, but not AP37, had yield penalties under normal paddy conditions in the field, whereas AP37 overexpressing lines, but not AP59, had enhanced yields under drought conditions in the field. The reduced yields of the overexpressing lines of AP59 were attributed to effects on spikelet development. This study emphasizes the point that it is important to characterize gene effects on yield under field conditions.
Mapping stress responses has provided new insights and identified gaps in our knowledge of abiotic stress responses
From a meta-analysis of drought-stress related papers from the last 15 years, a complex model for plant responses to drought stress was produced [ 12 ]. This model details the interactions of sugars, ROS/RNS, hormones (ABA, ethylene, auxins, cytokinins, salicylic acid, gibberellin and brassinosteroids) and nitrogen metabolism. It highlights the highly complex nature of stress responses.
From this review, we have constructed a simplified working model summarizing some of the known plant signaling responses to abiotic stress (Figure 2 ). Much of the signaling involves phosphorylation cascades that react quickly in the plant cell, emphasizing the need for proteomics data as well as transcriptomics data in future models. The PYR/PYL/RCAR-PP2C-SnRK2 pathway illustrates that protein phosphorylation and dephosphorylation are the most important factors in ABA signaling. Similar phosphorylation and dephosphorylation processes are involved in ethylene and other abiotic stress signaling pathways (Figure 2 ). Not all connections could be drawn in this two-dimensional figure without obscuring many other connections. For example, the interactions of ROS with abiotic stresses and hormones [ 32 ] are too complex to display here. In addition, the actual signaling response will be dependent upon the signaling pathway present in that organ, tissue or cell at the time of the response. One needs to use more sophisticated bioinformatics programs like Cytoscape [ 119 ] and its plug-ins to visualize the interactions comprehensively in two dimensional or three-dimensional space [ 120 ] or with time series views [ 121 ], which would allow these data to be viewed in four dimensions.
Although there are still some technological issues that must be solved to produce a complete picture of protein phosphorylation, several technologies have been developed for the large-scale analysis of phosphoproteins, known as 'phosphoproteomics' [ 122 ]. Mass spectrometry analyses have identified thousands of phosphoproteins in Arabidopsis, rice, and Medicago truncatula [ 123 – 125 ]. In addition, two studies have reported ABA-responsive changes in the phosphoproteome [ 126 , 127 ]. Phosphoproteomics analyses of mutants for abiotic stress signaling (e.g. PP2C or SnRK) will identify the relevant network of protein phosphorylation events in abiotic stress signaling.
Transcriptome analysis technologies have advanced to the point where high-through-put DNA sequencers and high-density microarrays such as tiling arrays are readily available. These technologies provide new opportunities to analyze noncoding RNAs and can clarify aspects of epigenetic regulation of gene expression [ 128 , 129 ]. Similar approaches [ 130 , 131 ] have revealed the global transcriptomes of plants exposed to abiotic stresses such as dehydration, cold, heat, high-salinity, osmotic stress, and ABA. These analyses indicate that these stresses increase or decrease transcript abundance from not only previously identified stress-responsive genes, but also from thousands of unannotated non-protein-coding regions. Matsui et al. [ 130 ] estimated that approximately 80% of previously unannotated upregulated transcripts arise from antisense strands of sense transcripts. There was a significant linear correlation between the expression ratios (stress-treated/untreated) of the sense transcripts and the ratios of the antisense transcripts. Interestingly, the data suggested that such stress-responsive antisense transcripts are derived from antisense strands of the stress-responsive genes, RD29A and CYP707A1 . Clearly, transcriptional regulation is far more complicated than we previously imagined. Whether or not such antisense transcripts have biological functions is an important issue that remains to be resolved.
Much more research is required in order to fully map plant responses to abiotic stress. The nature of the pathway responses will vary and is highly dependent on the species, organ, tissue, cell type, developmental stage of the plant, the stress or stresses affecting the plant, the level and duration of the stress. Despite the vast amount of research collected on abiotic stress in the last decade, there are still significant gaps in our knowledge. We still do not understand completely how plants perceive stress. We don't know all of the receptors and their sites of action (organs, tissues and cellular components). While we know a lot about downstream signaling (i.e. transcriptional pathways), we know very little about the primary signaling (i.e. proteomics). Most of the literature on abiotic stress responses in plants is based upon transcriptomics data rather than proteomics data. This is not surprising as transcriptomics technology is more advanced, easier to perform and less expensive. However, transcriptomics analyses are insufficient as there is an overall poor correlation of transcriptomics profiles with proteomics profiles [ 101 , 132 , 133 ] or enzyme activities [ 104 , 114 ]. There are only a few studies describing phosphorylation cascades and other post-translation modification activities in response to stress [ 134 ]. Recent efforts to map the hormone [ 126 , 127 ] and light-regulated [ 135 ] phosphorylomes are good first steps. Finally, we need better tools to facilitate systems biology analyses especially in the area of bioinformatics. Transcriptomics data can be collected in a matter of days or weeks, but the data analyses often take more than a year.
Conclusions
We have made great progress in understanding the responses of plants to abiotic stress. There are inherent physical, morphological and molecular limitations to the plant's ability to respond to stress. Systems biology approaches have given us a more holistic view of the molecular responses. Transcriptomics studies are well advanced, but proteomics analyses are lagging behind, especially the study of post-translational modifications. Plant responses to abiotic stress are dynamic and complex. The integration of multiple omics studies has revealed new areas of interactions and regulation. Time series experiments have revealed the kinetics of stress responses, identifying multiple response phases involving core sets of genes and condition-dependent changes. One consistent trend in response to abiotic stress is the early down regulation of energy metabolism and protein synthesis. This may indicate a conservation of energy by the plant and may reflect a shift from plant growth to protective mechanisms. In many examples presented in this review, ABA signaling mediates the plant responses to abiotic stress. Co-expression analyses are useful in that they have revealed key regulatory hubs that can be manipulated to produce different phenotypes. To get a comprehensive understanding of plant responses to abiotic stress, more extensive mapping of these responses at the organ, tissue and cellular level are needed. Such network analyses need to be extended to the proteomics and enzyme activities levels. Models need to be constructed and linked to phenotypic traits. The linkage of key regulatory hubs to phenotypic traits will allow for more rapid progress in the genetic manipulation and production of crop plants. Current progress is exemplified by the identification and validation of several key genes that improved stress tolerance of crops in the field. It is expected that progress in the plant sciences and systems biology will continue to accelerate in the near future.
Kitano H: Systems biology: a brief overview. Science. 2002, 295 (5560): 1662-1664. 10.1126/science.1069492.
PubMed CAS Google Scholar
Feist AM, Palsson BO: The growing scope of applications of genome-scale metabolic reconstructions using Escherichia coli. Nat Biotechnol. 2008, 26 (6): 659-667. 10.1038/nbt1401.
PubMed CAS PubMed Central Google Scholar
Bemis SM, Torii KU: Autonomy of cell proliferation and developmental programs during Arabidopsis aboveground organ morphogenesis. Dev Biol. 2007, 304 (1): 367-381. 10.1016/j.ydbio.2006.12.049.
Riefler M, Novak O, Strnad M, Schmulling T: Arabidopsis cytokinin receptor mutants reveal functions in shoot growth, leaf senescence, seed size, germination, root development, and cytokinin metabolism. Plant Cell. 2006, 18 (1): 40-54. 10.1105/tpc.105.037796.
Gibeaut DM, Hulett J, Cramer GR, Seemann JR: Maximal biomass of Arabidopsis thaliana using a simple, low-maintenance hydroponic method and favorable environmental conditions. Plant Physiol. 1997, 115: 317-319. 10.1104/pp.115.2.317.
Boyer JS: Plant productivity and environment. Science. 1982, 218 (4571): 443-448. 10.1126/science.218.4571.443.
Lobell DB, Schlenker W, Costa-Roberts J: Climate trends and global crop production since 1980. Science. 2011, 333 (6042): 616-620. 10.1126/science.1204531.
Skirycz A, Inze D: More from less: plant growth under limited water. Curr Opin Biotechnol. 2010, 21 (2): 197-203. 10.1016/j.copbio.2010.03.002.
Cramer GR: Abiotic stress & plant responses from the whole vine to the genes. Aust J Grape Wine Res. 2010, 16: 86-93.
CAS Google Scholar
Dinneny JR, Long TA, Wang JY, Jung JW, Mace D, Pointer S, Barron C, Brady SM, Schiefelbein J, Benfey PN: Cell identity mediates the response of Arabidopsis roots to abiotic stress. Science. 2008, 320 (5878): 942-945. 10.1126/science.1153795.
Tattersall EA, Grimplet J, Deluc L, Wheatley MD, Vincent D, Osborne C, Ergul A, Lomen E, Blank RR, Schlauch KA, Cushman JC, Cramer GR: Transcript abundance profiles reveal larger and more complex responses of grapevine to chilling compared to osmotic and salinity stress. Funct Integr Genomics. 2007, 7 (4): 317-333. 10.1007/s10142-007-0051-x.
Pinheiro C, Chaves MM: Photosynthesis and drought: can we make metabolic connections from available data?. J Exp Bot. 2011, 62 (3): 869-882. 10.1093/jxb/erq340.
Boyer JS: Evans Review: Cell wall biosynthesis and the molecular mechanism of plant enlargement. Funct Plant Biol. 2009, 36 (5): 383-394. 10.1071/FP09048.
Parent B, Hachez C, Redondo E, Simonneau T, Chaumont F, Tardieu F: Drought and abscisic acid effects on aquaporin content translate into changes in hydraulic conductivity and leaf growth rate: a trans-scale approach. Plant Physiol. 2009, 149 (4): 2000-2012. 10.1104/pp.108.130682.
Boursiac Y, Boudet J, Postaire O, Luu DT, Tournaire-Roux C, Maurel C: Stimulus-induced downregulation of root water transport involves reactive oxygen species-activated cell signalling and plasma membrane intrinsic protein internalization. Plant J. 2008, 56 (2): 207-218. 10.1111/j.1365-313X.2008.03594.x.
Vandeleur RK, Mayo G, Shelden MC, Gilliham M, Kaiser BN, Tyerman SD: The role of PIP aquaporins in water transport through roots: diurnal and drought stress responses reveal different strategies between isohydric and anisohydric cultivars of grapevine. Plant Physiol. 2008
Google Scholar
Nardini A, Lo GMA, Salleo S: Refilling embolized xylem conduits: is it a matter of phloem unloading?. Plant Sci. 2011, 180 (4): 604-611. 10.1016/j.plantsci.2010.12.011.
Hummel I, Pantin F, Sulpice R, Piques M, Rolland G, Dauzat M, Christophe A, Pervent M, Bouteille M, Stitt M, Gibon Y, Muller B: Arabidopsis plants acclimate to water deficit at low cost through changes of carbon usage: an integrated perspective using growth, metabolite, enzyme, and gene expression analysis. Plant Physiol. 2010, 154 (1): 357-372. 10.1104/pp.110.157008.
Cramer GR, Alberico GJ, Schmidt C: Leaf expansion limits dry matter accumulation of salt-stressed maize. Aust J Plant Physiol. 1994, 21: 663-674. 10.1071/PP9940663.
Nonami H, Wu YJ, Boyer JS: Decreased growth-induced water potential: primary cause of growth inhibition at low water potentials. Plant Physiol. 1997, 114 (2): 501-509.
Tang AC, Boyer JS: Growth-induced water potentials and the growth of maize leaves. J Exp Bot. 2002, 53 (368): 489-503. 10.1093/jexbot/53.368.489.
Good AG, Zaplachinski ST: The effects of drought stress on free amino acid accumulation and protein synthesis in brassica napus. Physiol Plant. 1994, 90 (1): 9-14. 10.1111/j.1399-3054.1994.tb02185.x.
Vincent D, Ergul A, Bohlman MC, Tattersall EA, Tillett RL, Wheatley MD, Woolsey R, Quilici DR, Joets J, Schlauch K, Schooley DA, Cushman JC, Cramer GR: Proteomic analysis reveals differences between Vitis vinifera L. cv. Chardonnay and cv. Cabernet Sauvignon and their responses to water deficit and salinity. J Exp Bot. 2007, 58 (7): 1873-1892. 10.1093/jxb/erm012.
Ben-Zioni A, Itai C, Vaadia Y: Water and salt stresses, kinetin and protein synthesis in tobacco leaves. Plant Physiol. 1967, 42: 361-365. 10.1104/pp.42.3.361.
Dhindsa RS, Cleland RE: Water stress and protein synthesis: I. Differential inhibition of protein synthesis. Plant Physiol. 1975, 55 (4): 778-781. 10.1104/pp.55.4.778.
Liu JX, Howell SH: Endoplasmic reticulum protein quality control and its relationship to environmental stress responses in plants. Plant Cell. 2010, 22 (9): 2930-2942. 10.1105/tpc.110.078154.
Cramer GR, Ergul A, Grimplet J, Tillett RL, Tattersall EA, Bohlman MC, Vincent D, Sonderegger J, Evans J, Osborne C, Quilici D, Schlauch KA, Schooley DA, Cushman JC: Water and salinity stress in grapevines: early and late changes in transcript and metabolite profiles. Funct Integr Genomics. 2007, 7 (2): 111-134. 10.1007/s10142-006-0039-y.
Kilian J, Whitehead D, Horak J, Wanke D, Weinl S, Batistic O, D'Angelo C, Bornberg-Bauer E, Kudla J, Harter K: The AtGenExpress global stress expression data set: protocols, evaluation and model data analysis of UV-B light, drought and cold stress responses. Plant J. 2007, 50 (2): 347-363. 10.1111/j.1365-313X.2007.03052.x.
Takahashi S, Seki M, Ishida J, Satou M, Sakurai T, Narusaka M, Kamiya A, Nakajima M, Enju A, Akiyama K, Yamaguchi-Shinozaki K, Shinozaki K: Monitoring the expression profiles of genes induced by hyperosmotic, high salinity, and oxidative stress and abscisic acid treatment in Arabidopsis cell culture using a full-length cDNA microarray. Plant Mol Biol. 2004, 56 (1): 29-55. 10.1007/s11103-004-2200-0.
Molassiotis A, Fotopoulos V: Oxidative and nitrosative signaling in plants: two branches in the same tree?. Plant Signal Behav. 2011, 6 (2): 210-214. 10.4161/psb.6.2.14878.
Wilkinson S, Davies WJ: Drought, ozone, ABA and ethylene: new insights from cell to plant to community. Plant Cell Environ. 2009
Mittler R, Vanderauwera S, Suzuki N, Miller G, Tognetti VB, Vandepoele K, Gollery M, Shulaev V, Van BF: ROS signaling: the new wave?. Trends Plant Sci. 2011, 16 (6): 300-309. 10.1016/j.tplants.2011.03.007.
Goda H, Sasaki E, Akiyama K, Maruyama-Nakashita A, Nakabayashi K, Li W, Ogawa M, Yamauchi Y, Preston J, Aoki K, Kiba T, Takatsuto S, Fujioka S, Asami T, Nakano T, Kato H, Mizuno T, Sakakibara H, Yamaguchi S, Nambara E, Kamiya Y, Takahashi H, Hirai MY, Sakurai T, Shinozaki K, Saito K, Yoshida S, Shimada Y: The AtGenExpress hormone and chemical treatment data set: experimental design, data evaluation, model data analysis and data access. Plant J. 2008, 55 (3): 526-542. 10.1111/j.1365-313X.2008.03510.x.
Hubbard KE, Nishimura N, Hitomi K, Getzoff ED, Schroeder JI: Early abscisic acid signal transduction mechanisms: newly discovered components and newly emerging questions. Genes Dev. 2010, 24 (16): 1695-1708. 10.1101/gad.1953910.
Kim TH, Bohmer M, Hu H, Nishimura N, Schroeder JI: Guard cell signal transduction network: advances in understanding abscisic acid, CO2, and Ca2+ signaling. Annu Rev Plant Biol. 2010, 61: 561-591. 10.1146/annurev-arplant-042809-112226.
Chinnusamy V, Gong Z, Zhu JK: Abscisic acid-mediated epigenetic processes in plant development and stress responses. J Integr Plant Biol. 2008, 50 (10): 1187-1195. 10.1111/j.1744-7909.2008.00727.x.
Umezawa T: Systems biology approaches to abscisic acid signaling. J Plant Res. 2011, 124 (4): 539-548. 10.1007/s10265-011-0418-x.
Ma Y, Szostkiewicz I, Korte A, Moes D, Yang Y, Christmann A, Grill E: Regulators of PP2C phosphatase activity function as abscisic acid sensors. Science. 2009, 324 (5930): 1064-1068.
Park SY, Fung P, Nishimura N, Jensen DR, Fujii H, Zhao Y, Lumba S, Santiago J, Rodrigues A, Chow TF, Alfred SE, Bonetta D, Finkelstein R, Provart NJ, Desveaux D, Rodriguez PL, McCourt P, Zhu JK, Schroeder JI, Volkman BF, Cutler SR: Abscisic acid inhibits type 2C protein phosphatases via the PYR/PYL family of START proteins. Science. 2009, 324 (5930): 1068-1071.
Leung J, Giraudat J: Abscisic acid signal transduction. Annu Rev Plant Physiol Plant Mol Biol. 1998, 49: 199-222. 10.1146/annurev.arplant.49.1.199.
Yoshida R, Hobo T, Ichimura K, Mizoguchi T, Takahashi F, Aronso J, Ecker JR, Shinozaki K: ABA-activated SnRK2 protein kinase is required for dehydration stress signaling in Arabidopsis. Plant Cell Physiol. 2002, 43 (12): 1473-1483. 10.1093/pcp/pcf188.
Mustilli AC, Merlot S, Vavasseur A, Fenzi F, Giraudat J: Arabidopsis OST1 protein kinase mediates the regulation of stomatal aperture by abscisic acid and acts upstream of reactive oxygen species production. Plant Cell. 2002, 14 (12): 3089-3099. 10.1105/tpc.007906.
Umezawa T, Nakashima K, Miyakawa T, Kuromori T, Tanokura M, Shinozaki K, Yamaguchi-Shinozaki K: Molecular basis of the core regulatory network in aba responses: sensing, signaling and transport. Plant Cell Physiol. 2010, 51 (11): 1821-1839. 10.1093/pcp/pcq156.
Yamaguchi-Shinozaki K, Shinozaki K: Transcriptional regulatory networks in cellular responses and tolerance to dehydration and cold stresses. Annu Rev Plant Biol. 2006, 57: 781-803. 10.1146/annurev.arplant.57.032905.105444.
Fujita Y, Fujita M, Satoh R, Maruyama K, Parvez MM, Seki M, Hiratsu K, Ohme-Takagi M, Shinozaki K, Yamaguchi-Shinozaki K: AREB1 is a transcription activator of novel ABRE-dependent ABA signaling that enhances drought stress tolerance in Arabidopsis. Plant Cell. 2005, 17 (12): 3470-3488. 10.1105/tpc.105.035659.
Kang JY, Choi HI, Im MY, Kim SY: Arabidopsis basic leucine zipper proteins that mediate stress-responsive abscisic Acid signaling. Plant Cell. 2002, 14 (2): 343-357. 10.1105/tpc.010362.
Yoshida T, Fujita Y, Sayama H, Maruyama K, Mizoi J, Shinozaki K, Yamaguchi-Shinozaki K: AREB1, AREB2, and ABF3 are master transcription factors that cooperatively regulate ABRE-dependent ABA signaling involved in drought stress tolerance and require ABA for full activation. Plant J. 2010, 61 (4): 672-685. 10.1111/j.1365-313X.2009.04092.x.
Fowler S, Thomashow MF: Arabidopsis transcriptome profiling indicates that multiple regulatory pathways are activated during cold acclimation in addition to the CBF cold response pathway. Plant Cell. 2002, 14 (8): 1675-1690. 10.1105/tpc.003483.
Maruyama K, Sakuma Y, Kasuga M, Ito Y, Seki M, Goda H, Shimada Y, Yoshida S, Shinozaki K, Yamaguchi-Shinozaki K: Identification of cold-inducible downstream genes of the Arabidopsis DREB1A/CBF3 transcriptional factor using two microarray systems. Plant J. 2004, 38 (6): 982-993. 10.1111/j.1365-313X.2004.02100.x.
Sakuma Y, Maruyama K, Osakabe Y, Qin F, Seki M, Shinozaki K, Yamaguchi-Shinozaki K: Functional analysis of an Arabidopsis transcription factor, DREB2A, involved in drought-responsive gene expression. Plant Cell. 2006, 18 (5): 1292-1309. 10.1105/tpc.105.035881.
Stepanova AN, Alonso JM: Ethylene signaling and response: where different regulatory modules meet. Curr Opin Plant Biol. 2009, 12 (5): 548-555. 10.1016/j.pbi.2009.07.009.
Yoo SD, Cho Y, Sheen J: Emerging connections in the ethylene signaling network. Trends Plant Sci. 2009, 14 (5): 270-279. 10.1016/j.tplants.2009.02.007.
Morgan PW, Drew MC: Ethylene and plant responses to stress. Physiol Plant. 1997, 100 (3): 620-630. 10.1111/j.1399-3054.1997.tb03068.x.
Zhang M, Leng P, Zhang G, Li X: Cloning and functional analysis of 9-cis-epoxycarotenoid dioxygenase (NCED) genes encoding a key enzyme during abscisic acid biosynthesis from peach and grape fruits. J Plant Physiol. 2009, 166 (12): 1241-1252. 10.1016/j.jplph.2009.01.013.
Sun L, Zhang M, Ren J, Qi J, Zhang G, Leng P: Reciprocity between abscisic acid and ethylene at the onset of berry ripening and after harvest. BMC Plant Biol. 2010, 10: 257-10.1186/1471-2229-10-257.
PubMed PubMed Central Google Scholar
Ophir R, Pang X, Halaly T, Venkateswari J, Lavee S, Galbraith D, Or E: Gene-expression profiling of grape bud response to two alternative dormancy-release stimuli expose possible links between impaired mitochondrial activity, hypoxia, ethylene-ABA interplay and cell enlargement. Plant Mol Biol. 2009, 71 (4-5): 403-423. 10.1007/s11103-009-9531-9.
Petranovic D, Tyo K, Vemuri GN, Nielsen J: Prospects of yeast systems biology for human health: integrating lipid, protein and energy metabolism. FEMS Yeast Res. 2010, 10 (8): 1046-1059. 10.1111/j.1567-1364.2010.00689.x.
Zaborske JM, Wu X, Wek RC, Pan T: Selective control of amino acid metabolism by the GCN2 eIF2 kinase pathway in Saccharomyces cerevisiae. BMC Biochem. 2010, 11: 29-10.1186/1471-2091-11-29.
Staschke KA, Dey S, Zaborske JM, Palam LR, McClintick JN, Pan T, Edenberg HJ, Wek RC: Integration of general amino acid control and target of rapamycin (TOR) regulatory pathways in nitrogen assimilation in yeast. J Biol Chem. 2010, 285 (22): 16893-16911. 10.1074/jbc.M110.121947.
Smeekens S, Ma J, Hanson J, Rolland F: Sugar signals and molecular networks controlling plant growth. Curr Opin Plant Biol. 2010, 13 (3): 274-279.
Hey SJ, Byrne E, Halford NG: The interface between metabolic and stress signalling. Ann Bot. 2010, 105 (2): 197-203. 10.1093/aob/mcp285.
Deprost D, Yao L, Sormani R, Moreau M, Leterreux G, Nicolai M, Bedu M, Robaglia C, Meyer C: The Arabidopsis TOR kinase links plant growth, yield, stress resistance and mRNA translation. EMBO Rep. 2007, 8 (9): 864-870. 10.1038/sj.embor.7401043.
Lageix S, Lanet E, Pouch-Pelissier MN, Espagnol MC, Robaglia C, Deragon JM, Pelissier T: Arabidopsis eIF2alpha kinase GCN2 is essential for growth in stress conditions and is activated by wounding. BMC Plant Biol. 2008, 8: 134-10.1186/1471-2229-8-134.
Baena-Gonzalez E, Rolland F, Thevelein JM, Sheen J: A central integrator of transcription networks in plant stress and energy signalling. Nature. 2007, 448 (7156): 938-942. 10.1038/nature06069.
Baena-Gonzalez E, Sheen J: Convergent energy and stress signaling. Trends Plant Sci. 2008, 13 (9): 474-482. 10.1016/j.tplants.2008.06.006.
Jossier M, Bouly JP, Meimoun P, Arjmand A, Lessard P, Hawley S, Grahame HD, Thomas M: SnRK1 (SNF1-related kinase 1) has a central role in sugar and ABA signalling in Arabidopsis thaliana. Plant J. 2009, 59 (2): 316-328. 10.1111/j.1365-313X.2009.03871.x.
Obertello M, Krouk G, Katari MS, Runko SJ, Coruzzi GM: Modeling the global effect of the basic-leucine zipper transcription factor 1 (bZIP1) on nitrogen and light regulation in Arabidopsis. BMC Systems Biol. 2010, 4: 111-10.1186/1752-0509-4-111.
Kang SG, Price J, Lin PC, Hong JC, Jang JC: The arabidopsis bZIP1 transcription factor is involved in sugar signaling, protein networking, and DNA binding. Mol Plant. 2010, 3 (2): 361-373. 10.1093/mp/ssp115.
Osuna D, Usadel B, Morcuende R, Gibon Y, Blasing OE, Hohne M, Gunter M, Kamlage B, Trethewey R, Scheible WR, Stitt M: Temporal responses of transcripts, enzyme activities and metabolites after adding sucrose to carbon-deprived Arabidopsis seedlings. Plant J. 2007, 49 (3): 463-491. 10.1111/j.1365-313X.2006.02979.x.
Zhou L, Jang JC, Jones TL, Sheen J: Glucose and ethylene signal transduction crosstalk revealed by an Arabidopsis glucose-insensitive mutant. Proc Natl Acad Sci USA. 1998, 95 (17): 10294-10299. 10.1073/pnas.95.17.10294.
Arenas-Huertero F, Arroyo A, Zhou L, Sheen J, Leon P: Analysis of Arabidopsis glucose insensitive mutants, gin5 and gin6, reveals a central role of the plant hormone ABA in the regulation of plant vegetative development by sugar. Genes Dev. 2000, 14 (16): 2085-2096.
Finkelstein RR, Gibson SI: ABA and sugar interactions regulating development: cross-talk or voices in a crowd?. Curr Opin Plant Biol. 2002, 5 (1): 26-32. 10.1016/S1369-5266(01)00225-4.
Franco-Zorrilla JM, Martin AC, Leyva A, Paz-Ares J: Interaction between phosphate-starvation, sugar, and cytokinin signaling in Arabidopsis and the roles of cytokinin receptors CRE1/AHK4 and AHK3. Plant Physiol. 2005, 138 (2): 847-857. 10.1104/pp.105.060517.
Mita S, Suzuki-Fujii K, Nakamura K: Sugar-inducible expression of a gene for beta-amylase in Arabidopsis thaliana. Plant Physiol. 1995, 107 (3): 895-904. 10.1104/pp.107.3.895.
Thum KE, Shasha DE, Lejay LV, Coruzzi GM: Light- and carbon-signaling pathways. Modeling circuits of interactions. Plant Physiol. 2003, 132 (2): 440-452. 10.1104/pp.103.022780.
Cakir B, Agasse A, Gaillard C, Saumonneau A, Delrot S, Atanassova R: A grape ASR protein involved in sugar and abscisic acid signaling. Plant Cell. 2003, 15 (9): 2165-2180. 10.1105/tpc.013854.
Lecourieux F, Lecourieux D, Vignault C, Delrot S: A sugar inducible protein kinase, VvSK1, regulates hexose transport and sugar accumulation in grapevine cells. Plant Physiol. 2010, 52: 1096-1106.
Shen W, Reyes MI, Hanley-Bowdoin L: Arabidopsis protein kinases GRIK1 and GRIK2 specifically activate SnRK1 by phosphorylating its activation loop. Plant Physiol. 2009, 150 (2): 996-1005. 10.1104/pp.108.132787.
Tiessen A, Prescha K, Branscheid A, Palacios N, McKibbin R, Halford NG, Geigenberger P: Evidence that SNF1-related kinase and hexokinase are involved in separate sugar-signalling pathways modulating post-translational redox activation of ADP-glucose pyrophosphorylase in potato tubers. Plant J. 2003, 35 (4): 490-500. 10.1046/j.1365-313X.2003.01823.x.
Zheng Z, Xu X, Crosley RA, Greenwalt SA, Sun Y, Blakeslee B, Wang L, Ni W, Sopko MS, Yao C, Yau K, Burton S, Zhuang M, McCaskill DG, Gachotte D, Thompson M, Greene TW: The protein kinase SnRK2.6 mediates the regulation of sucrose metabolism and plant growth in Arabidopsis. Plant Physiol. 2010, 153 (1): 99-113. 10.1104/pp.109.150789.
Lenz T, Fischer JJ, Dreger M: Probing small molecule-protein interactions: A new perspective for functional proteomics. J Proteomics. 2011
Kaufmann K, Smaczniak C, de VS, Angenent GC, Karlova R: Proteomics insights into plant signaling and development. Proteomics. 2011, 11 (4): 744-755. 10.1002/pmic.201000418.
Usadel B, Obayashi T, Mutwil M, Giorgi FM, Bassel GW, Tanimoto M, Chow A, Steinhauser D, Persson S, Provart NJ: Co-expression tools for plant biology: opportunities for hypothesis generation and caveats. Plant Cell Environ. 2009, 32 (12): 1633-1651. 10.1111/j.1365-3040.2009.02040.x.
Hirai MY, Yano M, Goodenowe DB, Kanaya S, Kimura T, Awazuhara M, Arita M, Fujiwara T, Saito K: Integration of transcriptomics and metabolomics for understanding of global responses to nutritional stresses in Arabidopsis thaliana. Proc Natl Acad Sci USA. 2004, 101 (27): 10205-10210. 10.1073/pnas.0403218101.
Hirai MY, Sugiyama K, Sawada Y, Tohge T, Obayashi T, Suzuki A, Araki R, Sakurai N, Suzuki H, Aoki K, Goda H, Nishizawa OI, Shibata D, Saito K: Omics-based identification of Arabidopsis Myb transcription factors regulating aliphatic glucosinolate biosynthesis. Proc Natl Acad Sci USA. 2007, 104 (15): 6478-6483. 10.1073/pnas.0611629104.
Mao L, Van HJL, Dash S, Dickerson JA: Arabidopsis gene co-expression network and its functional modules. BMC Bioinformatics. 2009, 10: 346-10.1186/1471-2105-10-346.
Carrera J, Rodrigo G, Jaramillo A, Elena SF: Reverse-engineering the Arabidopsis thaliana transcriptional network under changing environmental conditions. Genome Biol. 2009, 10 (9): R96.-
Lorenz WW, Alba R, Yu YS, Bordeaux JM, Simoes M, Dean JF: Microarray analysis and scale-free gene networks identify candidate regulators in drought-stressed roots of loblolly pine (P. taeda L.). BMC Genomics. 2011, 12: 264-10.1186/1471-2164-12-264.
Weston DJ, Gunter LE, Rogers A, Wullschleger SD: Connecting genes, coexpression modules, and molecular signatures to environmental stress phenotypes in plants. BMC Systems Biol. 2008, 2: 16-10.1186/1752-0509-2-16.
Weston DJ, Karve AA, Gunter LE, Jawdy SS, Yang X, Allen SM, Wullschleger SD: Comparative physiology and transcriptional networks underlying the heat shock response in Populus trichocarpa, Arabidopsis thaliana and Glycine max. Plant Cell Environ. 2011
Sun X, Zou Y, Nikiforova V, Kurths J, Walther D: The complexity of gene expression dynamics revealed by permutation entropy. BMC Bioinformatics. 2010, 11: 607-10.1186/1471-2105-11-607.
Vanderauwera S, Zimmermann P, Rombauts S, Vandenabeele S, Langebartels C, Gruissem W, Inze D, Van BF: Genome-wide analysis of hydrogen peroxide-regulated gene expression in Arabidopsis reveals a high light-induced transcriptional cluster involved in anthocyanin biosynthesis. Plant Physiol. 2005, 139 (2): 806-821. 10.1104/pp.105.065896.
Tognetti VB, Van AO, Morreel K, Vandenbroucke K, van dCB, De CI, Chiwocha S, Fenske R, Prinsen E, Boerjan W, Genty B, Stubbs KA, Inze D, Van BF: Perturbation of indole-3-butyric acid homeostasis by the UDP-glucosyltransferase UGT74E2 modulates Arabidopsis architecture and water stress tolerance. Plant Cell. 2010, 22 (8): 2660-2679. 10.1105/tpc.109.071316.
Vanderauwera S, De BM, Van dSN, van dCB, Metzlaff M, Van BF: Silencing of poly(ADP-ribose) polymerase in plants alters abiotic stress signal transduction. Proc Natl Acad Sci USA. 2007, 104 (38): 15150-15155. 10.1073/pnas.0706668104.
De Block M, Verduyn C, De Brouwer D, Cornelissen M: Poly(ADP-ribose) polymerase in plants affects energy homeostasis, cell death and stress tolerance. Plant J. 2005, 41 (1): 95-106.
Kusano M, Tohge T, Fukushima A, Kobayashi M, Hayashi N, Otsuki H, Kondou Y, Goto H, Kawashima M, Matsuda F, Niida R, Matsui M, Saito K, Fernie AR: Metabolomics reveals comprehensive reprogramming involving two independent metabolic responses of Arabidopsis to UV-B light. Plant J. 2011, 67 (2): 354-369. 10.1111/j.1365-313X.2011.04599.x.
Wilkins O, Brautigam K, Campbell MM: Time of day shapes Arabidopsis drought transcriptomes. Plant J. 2010, 63 (5): 715-727. 10.1111/j.1365-313X.2010.04274.x.
Raj S, Brautigam K, Hamanishi ET, Wilkins O, Thomas BR, Schroeder W, Mansfield SD, Plant AL, Campbell MM: Clone history shapes Populus drought responses. Proc Natl Acad Sci USA. 2011
Grimplet J, Deluc LG, Tillett RL, Wheatley MD, Schlauch KA, Cramer GR, Cushman JC: Tissue-specific mRNA expression profiling in grape berry tissues. BMC Genomics. 2007, 8: 187-10.1186/1471-2164-8-187.
Deluc LG, Quilici DR, Decendit A, Grimplet J, Wheatley MD, Schlauch KA, Merillon JM, Cushman JC, Cramer GR: Water deficit alters differentially metabolic pathways affecting important flavor and quality traits in grape berries of Cabernet Sauvignon and Chardonnay. BMC Genomics. 2009, 10: 212-10.1186/1471-2164-10-212.
Grimplet J, Wheatley MD, Jouira HB, Deluc LG, Cramer GR, Cushman JC: Proteomic and selected metabolite analysis of grape berry tissues under well watered and water-deficit stress conditions. Proteomics. 2009, 9: 2503-2528. 10.1002/pmic.200800158.
Deluc LG, Decendit A, Papastamoulis Y, Merillon JM, Cushman JC, Cramer GR: Water Deficit Increases Stilbene Metabolism in Cabernet Sauvignon Berries. J Agric Food Chem. 2011, 59 (1): 289-297. 10.1021/jf1024888.
Zamboni A, Di Carli M, Guzzo F, Stocchero M, Zenoni S, Ferrarini A, Tononi P, Toffali K, Desiderio A, Lilley KS, Pe ME, Benvenuto E, Delledonne M, Pezzotti M: Identification of putative stage-specific grapevine berry biomarkers and omics data integration into networks. Plant Physiol. 2010, 154 (3): 1439-1459. 10.1104/pp.110.160275.
Usadel B, Blasing OE, Gibon Y, Poree F, Hohne M, Gunter M, Trethewey R, Kamlage B, Poorter H, Stitt M: Multilevel genomic analysis of the response of transcripts, enzyme activities and metabolites in Arabidopsis rosettes to a progressive decrease of temperature in the non-freezing range. Plant Cell Environ. 2008, 31 (4): 518-547. 10.1111/j.1365-3040.2007.01763.x.
Caldana C, Degenkolbe T, Cuadros-Inostroza A, Klie S, Sulpice R, Leisse A, Steinhauser D, Fernie AR, Willmitzer L, Hannah MA: High-density kinetic analysis of the metabolomic and transcriptomic response of Arabidopsis to eight environmental conditions. Plant J. 2011
Yun KY, Park MR, Mohanty B, Herath V, Xu F, Mauleon R, Wijaya E, Bajic VB, Bruskiewich R, de LRBG: Transcriptional regulatory network triggered by oxidative signals configures the early response mechanisms of japonica rice to chilling stress. BMC Plant Biol. 2010, 10: 16-10.1186/1471-2229-10-16.
Urano K, Maruyama K, Ogata Y, Morishita Y, Takeda M, Sakurai N, Suzuki H, Saito K, Shibata D, Kobayashi M, Yamaguchi-Shinozaki K, Shinozaki K: Characterization of the ABA-regulated global responses to dehydration in Arabidopsis by metabolomics. Plant J. 2009, 7: 1065-1078.
Cook D, Fowler S, Fiehn O, Thomashow MF: A prominent role for the CBF cold response pathway in configuring the low-temperature metabolome of Arabidopsis. Proc Natl Acad Sci USA. 2004, 101 (42): 15243-15248. 10.1073/pnas.0406069101.
Maruyama K, Takeda M, Kidokoro S, Yamada K, Sakuma Y, Urano K, Fujita M, Yoshiwara K, Matsukura S, Morishita Y, Sasaki R, Suzuki H, Saito K, Shibata D, Shinozaki K, Yamaguchi-Shinozaki K: Metabolic pathways involved in cold acclimation identified by integrated analysis of metabolites and transcripts regulated by DREB1A and DREB2A. Plant Physiol. 2009, 150 (4): 1972-1980. 10.1104/pp.109.135327.
Nakamichi N, Kusano M, Fukushima A, Kita M, Ito S, Yamashino T, Saito K, Sakakibara H, Mizuno T: Transcript profiling of an Arabidopsis PSEUDO RESPONSE REGULATOR arrhythmic triple mutant reveals a role for the circadian clock in cold stress response. Plant Cell Physiol. 2009, 50 (3): 447-462. 10.1093/pcp/pcp004.
Kaplan F, Kopka J, Haskell DW, Zhao W, Schiller KC, Gatzke N, Sung DY, Guy CL: Exploring the temperature-stress metabolome of Arabidopsis. Plant Physiol. 2004, 136 (4): 4159-4168. 10.1104/pp.104.052142.
Wienkoop S, Morgenthal K, Wolschin F, Scholz M, Selbig J, Weckwerth W: Integration of metabolomic and proteomic phenotypes: analysis of data covariance dissects starch and RFO metabolism from low and high temperature compensation response in Arabidopsis thaliana. Mol Cell Proteomics. 2008, 7 (9): 1725-1736. 10.1074/mcp.M700273-MCP200.
Salekdeh GH, Reynolds M, Bennett J, Boyer J: Conceptual framework for drought phenotyping during molecular breeding. Trends Plant Sci. 2009, 14 (9): 488-496. 10.1016/j.tplants.2009.07.007.
Armengaud P, Sulpice R, Miller AJ, Stitt M, Amtmann A, Gibon Y: Multilevel analysis of primary metabolism provides new insights into the role of potassium nutrition for glycolysis and nitrogen assimilation in Arabidopsis roots. Plant Physiol. 2009, 150 (2): 772-785. 10.1104/pp.108.133629.
Hu H, Dai M, Yao J, Xiao B, Li X, Zhang Q, Xiong L: Overexpressing a NAM, ATAF, and CUC (NAC) transcription factor enhances drought resistance and salt tolerance in rice. Proc Natl Acad Sci USA. 2006, 103 (35): 12987-12992. 10.1073/pnas.0604882103.
Xiao B, Huang Y, Tang N, Xiong L: Over-expression of a LEA gene in rice improves drought resistance under the field conditions. TAG Theoretical and applied genetics Theoretische und angewandte Genetik. 2007, 115 (1): 35-46.
Nelson DE, Repetti PP, Adams TR, Creelman RA, Wu J, Warner DC, Anstrom DC, Bensen RJ, Castiglioni PP, Donnarummo MG, Hinchey BS, Kumimoto RW, Maszle DR, Canales RD, Krolikowski KA, Dotson SB, Gutterson N, Ratcliffe OJ, Heard JE: Plant nuclear factor Y (NF-Y) B subunits confer drought tolerance and lead to improved corn yields on water-limited acres. Proc Natl Acad Sci USA. 2007, 104 (42): 16450-16455. 10.1073/pnas.0707193104.
Oh SJ, Kim YS, Kwon CW, Park HK, Jeong JS, Kim JK: Overexpression of the transcription factor AP37 in rice improves grain yield under drought conditions. Plant Physiol. 2009, 150 (3): 1368-1379. 10.1104/pp.109.137554.
Shannon P, Markiel A, Ozier O, Baliga NS, Wang JT, Ramage D, Amin N, Schwikowski B, Ideker T: Cytoscape: a software environment for integrated models of biomolecular interaction networks. Genome Res. 2003, 13 (11): 2498-2504. 10.1101/gr.1239303.
Audenaert P, Van PT, Brondel F, Pickavet M, Demeester P, Van dPY, Michoel T: CyClus3D: a Cytoscape plugin for clustering network motifs in integrated networks. Bioinformatics. 2011, 27 (11): 1587-1588. 10.1093/bioinformatics/btr182.
Xia T, Hemert JV, Dickerson JA: OmicsAnalyzer: a Cytoscape plug-in suite for modeling omics data. Bioinformatics. 2010, 26 (23): 2995-2996. 10.1093/bioinformatics/btq583.
Schulze WX: Proteomics approaches to understand protein phosphorylation in pathway modulation. Curr Opin Plant Biol. 2010, 13 (3): 280-287.
Sugiyama N, Nakagami H, Mochida K, Daudi A, Tomita M, Shirasu K, Ishihama Y: Large-scale phosphorylation mapping reveals the extent of tyrosine phosphorylation in Arabidopsis. Mol Syst Biol. 2008, 4: 193-
Nakagami H, Sugiyama N, Mochida K, Daudi A, Yoshida Y, Toyoda T, Tomita M, Ishihama Y, Shirasu K: Large-scale comparative phosphoproteomics identifies conserved phosphorylation sites in plants. Plant Physiol. 2010, 153 (3): 1161-1174. 10.1104/pp.110.157347.
de la Fuente van Bentem S, Anrather D, Roitinger E, Djamei A, Hufnagl T, Barta A, Csaszar E, Dohnal I, Lecourieux D, Hirt H: Phosphoproteomics reveals extensive in vivo phosphorylation of Arabidopsis proteins involved in RNA metabolism. Nucleic Acids Res. 2006, 34 (11): 3267-3278. 10.1093/nar/gkl429.
Chen Y, Hoehenwarter W, Weckwerth W: Comparative analysis of phytohormone-responsive phosphoproteins in Arabidopsis thaliana using TiO2-phosphopeptide enrichment and mass accuracy precursor alignment. Plant J. 2010, 63 (1): 1-17.
Kline KG, Barrett-Wilt GA, Sussman MR: In planta changes in protein phosphorylation induced by the plant hormone abscisic acid. Proc Natl Acad Sci USA. 2010, 107 (36): 15986-15991. 10.1073/pnas.1007879107.
Zhang X, Yazaki J, Sundaresan A, Cokus S, Chan SW, Chen H, Henderson IR, Shinn P, Pellegrini M, Jacobsen SE, Ecker JR: Genome-wide high-resolution mapping and functional analysis of DNA methylation in arabidopsis. Cell. 2006, 126 (6): 1189-1201. 10.1016/j.cell.2006.08.003.
Gregory BD, Yazaki J, Ecker JR: Utilizing tiling microarrays for whole-genome analysis in plants. Plant J. 2008, 53 (4): 636-644.
Matsui A, Ishida J, Morosawa T, Mochizuki Y, Kaminuma E, Endo TA, Okamoto M, Nambara E, Nakajima M, Kawashima M, Satou M, Kim JM, Kobayashi N, Toyoda T, Shinozaki K, Seki M: Arabidopsis transcriptome analysis under drought, cold, high-salinity and ABA treatment conditions using a tiling array. Plant Cell Physiol. 2008, 49 (8): 1135-1149. 10.1093/pcp/pcn101.
Zeller G, Henz SR, Widmer CK, Sachsenberg T, Ratsch G, Weigel D, Laubinger S: Stress-induced changes in the Arabidopsis thaliana transcriptome analyzed using whole-genome tiling arrays. Plant J. 2009, 58 (6): 1068-1082. 10.1111/j.1365-313X.2009.03835.x.
Nie L, Wu G, Culley DE, Scholten JC, Zhang W: Integrative analysis of transcriptomic and proteomic data: challenges, solutions and applications. Crit Rev Biotechnol. 2007, 27 (2): 63-75. 10.1080/07388550701334212.
Bohmer M, Schroeder JI: Quantitative transcriptomic analysis of abscisic acid-induced and reactive oxygen species-dependent expression changes and proteomic profiling in Arabidopsis suspension cells. Plant J. 2011, 67 (1): 105-118. 10.1111/j.1365-313X.2011.04579.x.
Yang S, Vanderbeld B, Wan J, Huang Y: Narrowing down the targets: towards successful genetic engineering of drought-tolerant crops. Mol Plant. 2010, 3 (3): 469-490. 10.1093/mp/ssq016.
Reiland S, Finazzi G, Endler A, Willig A, Baerenfaller K, Grossmann J, Gerrits B, Rutishauser D, Gruissem W, Rochaix JD, Baginsky S: Comparative phosphoproteome profiling reveals a function of the STN8 kinase in fine-tuning of cyclic electron flow (CEF). Proc Natl Acad Sci USA. 2011.
Download references
Author information
Authors and affiliations.
Department of Biochemistry and Molecular Biology, Mail Stop 330, University of Nevada, Reno, Nevada, 89557, USA
Grant R Cramer
Gene Discovery Research Group, RIKEN Plant Science Center, 3-1-1 Koyadai, Tsukuba, 305-0074, Japan
Kaoru Urano & Kazuo Shinozaki
Univ. Bordeaux, ISVV, Ecophysiologie et Génomique Fonctionnelle de la Vigne, UMR 1287, Villenave d'Ornon, F-33882, France
Serge Delrot
Dipartimento di Biotecnologie, Università di Verona, Strada le Grazie 15, Verona, 37134, Italy
Mario Pezzotti
You can also search for this author in PubMed Google Scholar
Corresponding author
Correspondence to Grant R Cramer .
Additional information
Authors' contributions.
GRC contributed to all aspects of this manuscript. KU and KS contributed to the metabolomics and ABA signaling sections and Figure 2 . SD contributed to the introduction, ABA and sugar signaling sections. MP contributed to the time-series analyses section. All authors read and approved the final version of this manuscript.
Authors’ original submitted files for images
Below are the links to the authors’ original submitted files for images.
Authors’ original file for figure 1
Authors’ original file for figure 2, rights and permissions.
This article is published under license to BioMed Central Ltd. This is an Open Access article distributed under the terms of the Creative Commons Attribution License ( http://creativecommons.org/licenses/by/2.0 ), which permits unrestricted use, distribution, and reproduction in any medium, provided the original work is properly cited.
Reprints and permissions
About this article
Cite this article.
Cramer, G.R., Urano, K., Delrot, S. et al. Effects of abiotic stress on plants: a systems biology perspective. BMC Plant Biol 11 , 163 (2011). https://doi.org/10.1186/1471-2229-11-163
Download citation
Received : 05 September 2011
Accepted : 17 November 2011
Published : 17 November 2011
DOI : https://doi.org/10.1186/1471-2229-11-163
Share this article
Anyone you share the following link with will be able to read this content:
Sorry, a shareable link is not currently available for this article.
Provided by the Springer Nature SharedIt content-sharing initiative
- Abiotic Stress
- Reactive Nitrogen Species
- Antisense Transcript
- Abiotic Stress Response
BMC Plant Biology
ISSN: 1471-2229
- General enquiries: [email protected]
Abiotic stress responses in plants
Affiliations.
- 1 Shanghai Center for Plant Stress Biology, Center for Excellence in Molecular Plant Sciences, Chinese Academy of Sciences, Shanghai, China. [email protected].
- 2 Department of Plant Science and Landscape Architecture, University of Maryland, College Park, MD, USA.
- 3 State Key Laboratory of Plant Physiology and Biochemistry, College of Biological Sciences, China Agricultural University, Beijing, China.
- 4 Institute of Life Science and Green Development, School of Life Sciences, Hebei University, Baoding, China.
- 5 Shanghai Center for Plant Stress Biology, Center for Excellence in Molecular Plant Sciences, Chinese Academy of Sciences, Shanghai, China. [email protected].
- PMID: 34561623
- DOI: 10.1038/s41576-021-00413-0
Plants cannot move, so they must endure abiotic stresses such as drought, salinity and extreme temperatures. These stressors greatly limit the distribution of plants, alter their growth and development, and reduce crop productivity. Recent progress in our understanding of the molecular mechanisms underlying the responses of plants to abiotic stresses emphasizes their multilevel nature; multiple processes are involved, including sensing, signalling, transcription, transcript processing, translation and post-translational protein modifications. This improved knowledge can be used to boost crop productivity and agricultural sustainability through genetic, chemical and microbial approaches.
© 2021. Springer Nature Limited.
Publication types
- Research Support, Non-U.S. Gov't
- Atmosphere / chemistry
- Carbon Dioxide / metabolism
- Crops, Agricultural / genetics*
- Crops, Agricultural / growth & development
- Crops, Agricultural / metabolism
- Gene Expression Regulation, Plant
- Oxygen / metabolism
- Plants / genetics*
- Plants / metabolism
- Soil / chemistry
- Stress, Physiological / genetics*
- Temperature*
- Carbon Dioxide
EDITORIAL article
Editorial: advanced breeding for abiotic stress tolerance in crops.
- 1 Hainan Institute, Zhejiang University, Sanya, China
- 2 The Advanced Seed Institute, Zhejiang University, Hangzhou, China
- 3 College of Life Sciences, China Jiliang University, Hangzhou, China
Editorial on the Research Topic Advanced breeding for abiotic stress tolerance in crops
1 Introduction
Abiotic stress, including extreme temperature, salinity, drought, and other environmental pollution with excessive heavy metals, is regarded to be a global issue in agricultural systems that results in considerable yield and quality losses for crops ( Waadt et al., 2022 ). With the continued rise of the world’s population, it is crucial for sustainable agriculture and food security to develop advanced breeding strategies that effectively mitigate abiotic stress ( Zhang et al., 2022 ). Additionally, a multifaceted strategy is required for crops to enhance their ability to adapt to abiotic stress, including hormone modulation, plant enzymatic system activation, and stress gene expression ( Gong et al., 2020 ). Therefore, understanding how cereal crops react to abiotic stress is crucial ( Mittler et al., 2022 ). Figure out the important characteristics of abiotic stress and their underlying physiological, biochemical, and molecular bases (e.g., genetic, epigenetic, transcriptomic, and metabolomic) will extend our knowledge in breeding efforts to create abiotic stress-resistant crops ( Chang et al., 2020 ).
In this editorial, we set up a Research Topic of Advanced Breeding for Abiotic Stress Tolerance in Crops, which covers up-to-date scientific evidence and the potential for future research to improve our knowledge of the mechanisms that control the development of abiotic stress tolerance in the world’s major crop species. The following themes are included in this Research Topic: (a) Advanced crop breeding applications for increasing abiotic stress resistance in crops; (b) Novel plant growth regulators for enhancing abiotic stress tolerance in plants; (c) Metabolomic and molecular strategies to improve abiotic stress-resistance in crops; (d) Genetic mechanisms related to abiotic stress tolerance in plants and their related traits in plants by quantitative trait loci (QTL) mapping, genome-wide association (GWAS) investigation, or QTL-sequencing; (e) Epigenetic bases of abiotic stress resistance and their applications in crop breeding.
Despite significant advances in understanding the underlying mechanism of abiotic stress, there remain knowledge gaps in these areas, and our Research Topic aims to address these gaps. In the end, we accepted and published 20 articles (16 Original Research, 3 Review, and 1 Perspective) written by 158 researchers from seven different countries, e.g. Australia, United Kingdom, Italy, Greece, Tunisia, India, and China. Unfolded protein response in balancing plant growth and stress tolerance, current trends and insights on EMS mutagenesis application to studies on abiotic stress tolerance and plant development, and achieving abiotic stress tolerance in plants through antioxidative defense mechanisms were reviewed by Liu et al. , Chen et al. , and Mishra et al. A Perspective named ‘A Holistic and Sustainable Approach Linked to Drought Tolerance of Mediterranean’ was written by Trovato et al. The 16 Original Research articles will be divided into the following four parts for detailed interpretation.

2 Assay of gene family under abiotic stress
- Yang et al. included a thorough analysis of 56 LOR ( Lurp-One-Related ) genes in Brassica napus . Some reports found that LOR gene family members acted key functions in defense of Hyaloperonospora parasitica (Hpa) in Arabidopsis . This research improved our understanding of the Brassica napus LOR gene family and may help in the selection and identification of genes for stress-resistant breeding.
- Pang et al. discovered 9 members of the APX gene family in the pepper genome according to the conserved domain of the APX protein in Arabidopsis thaliana . The work discovered the activities of APX genes and provided information for further functional characterisation of CaAPX genes.
- Zhang et al. identified 104 NAC genes in Camellia sinensis . The thorough characterisation of Camellia sinensis NAC genes provided by the study may operate as a starting point for research into the molecular basis of CsNACs -mediated drought response.
- Wang et al. discovered the 65 potential bZIP genes in Lagenaria siceraria and described their gene structure, phylogenetic and orthologous connections, gene expression patterns in various tissues and cultivars. Through the use of RNA-Seq and RT-PCR, they have examined and confirmed the cold stress-responsive candidate bZIP genes in Lagenaria siceraria . This has resulted in the discovery of new information regarding the transcriptional control of bottle gourd bZIP family genes and their potential roles in the development of cold-tolerant varieties through breeding.
- Yu et al. found that ten OsSnRK2 genes were discovered in the rice genome distributed across seven chromosomes and grouped into three subfamilies. The results offered valuable details for comprehending the OsSnRK2 gene family and examining how it responds to drought, salt, and ABA treatment, particularly drought-salt joint stress.
3 Multiomics analysis under abiotic stress
- Xu L. et al. employed a genome-wide association study (GWAS) methodology using 90K single nucleotide polymorphisms (SNPs) in a panel of 329 wheat genotypes to identify the quantitative trait loci (QTL) for ARs and RCA. In breeding efforts, a number of genotypes that consistently performed well under various conditions can be utilized to create wheat varieties that can withstand waterlogging.
- Sun et al. discovered that during drought stress, rice plants’ dry matter mass, N content, and N accumulation all grew to varying degrees even when the same amount of N was applied. The findings showed that pathways involved in energy metabolism, such as the phosphotransferase system (PTS) and D-alanine metabolism, were enriched. In general, the work offers a theoretical foundation for enhancing rice’s drought tolerance and N use efficiency.
- Zhao et al. studied the distribution of Zhe-Maidong ( Ophiopogon japonicus ) roots reacting to Cd stress and the features of Cd transformation by growing Zhe-Maidong seedlings for 90 days in Cd-contaminated and uncontaminated soil. The findings may be useful in determining the features of Cd accumulation in Zhe-Maidong and may also serve as a bioinformatic starting point for research on gene functions and the screening of potential Cd-accumulation-related candidate genes.
- Gu et al. discovered that the rice gene OsSEH1 , which codes for nucleoporins, is a positive regulator for cold stress. The findings showed that wild type plants and osseh1 lines had hypersensitive phenotypes, indicating that OsSEH1 may influence cold tolerance via controlling ABA levels.
4 Exploration of underlying mechanism under abiotic stress
- Zhang et al. reported that OsWRKY76 positively controlled rice drought stress. Dehydration stress, exogenous MeJA, and PEG treatments can induce the expression of OsWRKY76 . The findings provided a new lead in the quest to understand the processes behind drought tolerance by suggesting that OsWRKY76 provides drought tolerance through OsbHLH148-mediated jasmonate signaling in rice.
- Xu J. et al. discovered that the oil-tolerant plant Mirabilis jalapa contained a mannanase ( MAN ) gene called MirMAN , which was cloned and heterologously produced in Arabidopsis . The study showed that MirMAN is a potentially significant target gene that may be further used to enhance plant tolerance to abiotic stress.
- Zhou et al. investigated the effects of high temperature on rice ( Oryza sativa ) starch metabolite and the higher reductions in BEI , BEIIa , BEIIb , and SSIVb expression when exposed to high temperature. The study found that by generating more starch-lipid complexes under high temperatures, the increased long chains of amylopectin and lipids may be the main cause of the enhanced RS content in mutants.
- Su et al. measured physiological-biochemical parameters and assessed the drought tolerance of various wheat cultivars. While demonstrating the possibility of TaPRX-2A overexpression in boosting drought tolerance during crop development efforts, the study offered insights into the processes of tolerance.
- Jiang et al. found that during the progressive drought and water recovery phases, the midday transpiration rate Tr (Tr m ) and daily transpiration (E) as well as plant growth of water recovery at θcri (WR_θcri) was slightly lower or comparable to those of well irrigation (CK), but those of water recovery under severe drought (WR_SD) were significantly lower than those of CK during water stress and did not recover after rehydration. According to the findings, irrigation balances strawberry growth and water use at a key soil water content.
5 Advanced techniques in abiotic stress research
- Shen et al. discovered that precisely introducing a regulatory element into a target gene’s 5’UTR might effectively boost the protein abundance of that gene in rice. The research offered a fresh method to control protein translation for crop breeding by demonstrating the viability of such in-locus editing to improve protein expression.
- Si et al. found that virus-induced gene silencing (VIGS) is continuously tracked by a visible gland in cotton. The enhanced VIGS technology will provide another tool for the quick functional identification of many genomes’ undiscovered genes.
It is crucial to comprehend how general and stress-specific response pathways interact since plants must deal with numerous abiotic stresses at once in their natural habitat. Similar to this, stomatal closure and ROS generation are examples of regulatory nodes at which plant responses to abiotic stimuli may also converge. These numerous reactions, as well as the crosstalk between them, maybe simultaneously triggered and result in additive, synergistic, or antagonistic effects, which could improve or impair stress resistance. In order to discover essential molecular targets for breeding stress-resistant crops in the field, it will be necessary to untangle plant responses to various stresses at the molecular level by using multiomics analysis and advanced techniques.
Author contributions
TZ: Formal Analysis, Software, Supervision, Writing – original draft. XW: Data curation, Funding acquisition, Investigation, Project administration, Writing – original draft. MJ: Conceptualization, Funding acquisition, Methodology, Validation, Writing – review & editing.
This Research Topic was supported by the Research Startup Funding from the Hainan Institute of Zhejiang University (0201-6602-A12203) received by MJ and Zhejiang Science and Technology Major Program on Agricultural New Variety (2021C02067-7) received by XW.
Acknowledgments
We extend our heartfelt thanks to all authors and reviewers for their invaluable input and contributions. Additionally, we are grateful to the Journal Committee for providing us the opportunity to develop this Research Topic.
Conflict of interest
The authors declare that the research was conducted in the absence of any commercial or financial relationships that could be construed as a potential conflict of interest.
Publisher’s note
All claims expressed in this article are solely those of the authors and do not necessarily represent those of their affiliated organizations, or those of the publisher, the editors and the reviewers. Any product that may be evaluated in this article, or claim that may be made by its manufacturer, is not guaranteed or endorsed by the publisher.
Chang, Y. N., Zhu, C., Jiang, J., Zhang, H., Zhu, J. K., Duan, C. G. (2020). Epigenetic regulation in plant abiotic stress responses. J. Integr. Plant Biol. 62 (5), 563–580. doi: 10.1111/jipb.12901
PubMed Abstract | CrossRef Full Text | Google Scholar
Gong, Z., Xiong, L., Shi, H., Yang, S., Herrera-Estrella, L. R., Xu, G., et al. (2020). Plant abiotic stress response and nutrient use efficiency. Sci. China Life Sci. 63 (5), 635–674. doi: 10.1007/s11427-020-1683-x
Mittler, R., Zandalinas, S. I., Fichman, Y., Van Breusegem, F. (2022). Reactive oxygen species signalling in plant stress responses. Nat. Rev. Mol. Cell Biol. 23 (10), 663–679. doi: 10.1038/s41580-022-00499-2
Waadt, R., Seller, C. A., Hsu, P. K., Takahashi, Y., Munemasa, S., Schroeder, J. I. (2022). Plant hormone regulation of abiotic stress responses. Nat. Rev. Mol. Cell Biol. 23 (10), 680–694. doi: 10.1038/s41580-022-00479-6
Zhang, H., Zhu, J., Gong, Z., Zhu, J. K. (2022). Abiotic stress responses in plants. Nat. Rev. Genet. 23 (2), 104–119. doi: 10.1038/s41576-021-00413-0
Keywords: crops, breeding, abiotic stress, gene family, multiomics analysis, mechanism, advanced techniques
Citation: Zhao T, Wu X and Jiang M (2023) Editorial: Advanced breeding for abiotic stress tolerance in crops. Front. Plant Sci. 14:1265339. doi: 10.3389/fpls.2023.1265339
Received: 22 July 2023; Accepted: 02 August 2023; Published: 09 August 2023.
Edited and Reviewed by:
Copyright © 2023 Zhao, Wu and Jiang. This is an open-access article distributed under the terms of the Creative Commons Attribution License (CC BY) . The use, distribution or reproduction in other forums is permitted, provided the original author(s) and the copyright owner(s) are credited and that the original publication in this journal is cited, in accordance with accepted academic practice. No use, distribution or reproduction is permitted which does not comply with these terms.
*Correspondence: Meng Jiang, [email protected]
† These authors have contributed equally to this work
Disclaimer: All claims expressed in this article are solely those of the authors and do not necessarily represent those of their affiliated organizations, or those of the publisher, the editors and the reviewers. Any product that may be evaluated in this article or claim that may be made by its manufacturer is not guaranteed or endorsed by the publisher.

An official website of the United States government
The .gov means it’s official. Federal government websites often end in .gov or .mil. Before sharing sensitive information, make sure you’re on a federal government site.
The site is secure. The https:// ensures that you are connecting to the official website and that any information you provide is encrypted and transmitted securely.
- Publications
- Account settings
Preview improvements coming to the PMC website in October 2024. Learn More or Try it out now .
- Advanced Search
- Journal List
- Front Plant Sci
Mechanisms Regulating the Dynamics of Photosynthesis Under Abiotic Stresses
Izhar muhammad.
1 State Key Laboratory of Crop Stress Biology for Arid Areas, College of Agronomy, Northwest A&F University, Yangling, China
2 State Key Laboratory of Crop Stress Biology for Arid Areas, College of Life Sciences, Northwest A&F University, Yangling, China
Abdullah Shalmani
Muhammad ali.
3 Department of Horticulture, Zhejiang University, Hangzhou, China
Qing-Hua Yang
Husain ahmad.
4 College of Horticulture, Northwest A&F University, Yangling, China
Feng Bai Li
Photosynthesis sustains plant life on earth and is indispensable for plant growth and development. Factors such as unfavorable environmental conditions, stress regulatory networks, and plant biochemical processes limits the photosynthetic efficiency of plants and thereby threaten food security worldwide. Although numerous physiological approaches have been used to assess the performance of key photosynthetic components and their stress responses, though, these approaches are not extensive enough and do not favor strategic improvement of photosynthesis under abiotic stresses. The decline in photosynthetic capacity of plants due to these stresses is directly associated with reduction in yield. Therefore, a detailed information of the plant responses and better understanding of the photosynthetic machinery could help in developing new crop plants with higher yield even under stressed environments. Interestingly, cracking of signaling and metabolic pathways, identification of some key regulatory elements, characterization of potential genes, and phytohormone responses to abiotic factors have advanced our knowledge related to photosynthesis. However, our understanding of dynamic modulation of photosynthesis under dramatically fluctuating natural environments remains limited. Here, we provide a detailed overview of the research conducted on photosynthesis to date, and highlight the abiotic stress factors (heat, salinity, drought, high light, and heavy metal) that limit the performance of the photosynthetic machinery. Further, we reviewed the role of transcription factor genes and various enzymes involved in the process of photosynthesis under abiotic stresses. Finally, we discussed the recent progress in the field of biodegradable compounds, such as chitosan and humic acid, and the effect of melatonin (bio-stimulant) on photosynthetic activity. Based on our gathered researched data set, the logical concept of photosynthetic regulation under abiotic stresses along with improvement strategies will expand and surely accelerate the development of stress tolerance mechanisms, wider adaptability, higher survival rate, and yield potential of plant species.
Introduction
Although multiple physiological, biochemical, and molecular processes collaboratively define plant productivity, where the stable photosynthetic performance is thought to be essential for healthy plant growth and development (Ashraf and Harris, 2013 ; Gururani et al., 2015 ; Nguyen et al., 2018 ; Sharma et al., 2020 ). Photosynthesis is a remarkable process, which is not only confined to the leaves of green plants but also occurs in young developing embryos of aquatic and land plants as well as in bacteria (Taiz and Zeiger, 2010 ; Pan et al., 2012 ). Photosynthesis fuels a number of metabolic processes by triggering the process of conversion of light energy into chemical energy (Chen et al., 2018 ; Demmig-Adams et al., 2018 ). Chloroplast is the houses of both the light and dark reactions of photosynthesis, and is highly responsive to abiotic stresses, heavy metal toxicity, nutrient deficiency or toxicity, hypoxia or anoxia, ultraviolet (UV) radiation, light intensity fluctuations (Mu et al., 2016 , 2017 ; Sharma et al., 2016a , 2019 ; Kaur et al., 2017 ; Demmig-Adams et al., 2018 ; Kohli et al., 2018 ; Paunov et al., 2018 ; Soares et al., 2018 ; Yadav et al., 2018 ). Abiotic stresses also have a negative impact on photosystem I (PSI) and PS II, electron transport chain (ETC), and chlorophyll (Chl) biosynthesis (Xia et al., 2006 ; Efeoglu and Terzioglu, 2009 ; Kalaji et al., 2016 ; Sharma et al., 2016b ). Additionally, abiotic stresses reduce stomatal conductance, inducing oxidative stress, which decreases the activity of ribulose-1,5-bisphosphate carboxylase/oxygenase (Rubisco) and obstructs the process of photosynthesis (Allen and Ort, 2001 ; Crafts-Brandner and Salvucci, 2004 ; Chaves et al., 2009 ; Zhang et al., 2014 ; Kohli et al., 2017 ). Reactive oxygen species (ROS) are synthesized in various compartments including plastids, mitochondria, peroxisomes, and the apoplast via different pathways and is controlled by the ROS gene network (Mittler et al., 2004 ). In addition, each cellular compartment controls its own ROS homeostasis, where different ROS levels in various compartments generate a particular ROS signature. Further, different ROS signature resulted from unlike abiotic stresses and/or combination of different abiotic stresses are decoded via different ROS sensors and thereafter initiate stress-specific signal in the affecting plant. Previous reports stated that ROS production occurs in chloroplasts within the ETCs of PSI and PSII during light reactions, and is enhanced when carbon dioxide (CO 2 ) supply is restricted and ATP synthesis is impaired (Takahashi and Murata, 2008 ; Nishiyama and Murata, 2014 ; Noctor et al., 2014 ; Tikkanen et al., 2014 ). The generation of ROS under normal conditions are the byproducts of metabolic pathways such as photosynthesis, respiration, and photorespiration, while over production of reactive oxygen species (ROS), such as hydrogen peroxide (H 2 O 2 ), singlet oxygen ( 1 O 2 ), the superoxide ( O 2 - ), and hydroxyl (HO . ) radicals, is a general feature under abiotic stresses (Anjum et al., 2016a , 2017 ; Soares et al., 2016b ; Guo et al., 2017a ; Czarnocka and Karpiński, 2018 ; Kaur et al., 2019 ; Sharma et al., 2019 ). There are two major sources of ROS during abiotic stresses, metabolic ROS [generated as a consequence of disruptions in metabolic activity (Miller et al., 2010 )] and signaling ROS [produced for signaling in response of the abiotic stress-response signal transduction network (Mittler et al., 2012 ; Tikkanen et al., 2014 )]. The enhanced level of intracellular ROS within the cells are mitigated by ROS detoxifying proteins such as superoxide dismutase (SOD), ascorbate peroxidase (APX), catalase (CAT), glutathione peroxidase (GPX), and peroxiredoxin (PRX) (Mittler et al., 2004 ), and ascorbate or glutathione (GSH) that are present in almost all subcellular compartments (Takahashi and Murata, 2008 ; Schmutz et al., 2010 ; Upadhyaya et al., 2011 ). However, ROS-scavenging systems is not that much efficient to completely remove intracellular ROS, therefore to increase abiotic stress tolerance, genetic manipulation of antioxidants and ROS-scavenging enzymes may be more appropriate approach (Tóth et al., 2011 ).
Plants adopt several mechanisms for photoprotection, like photorespiration (Streb et al., 2005 ), cyclic electron flow (Huang et al., 2015b ), alternative electron flow (Laureau et al., 2013 ), and antioxidant systems (Streb et al., 2003 ). Photorespiration consumes excess NADPH and, thus, alleviates its over-accumulation on the acceptor side of PSI, preventing the over-reduction of photosynthetic electron chains (Huang et al., 2015a ). For example, the alpine herb, Ranunculus glacialis , excess electrons are transferred to oxygen when photorespiration is blocked, due to a high content of plastid terminal oxidase (PTOX). This PTOX has the capacity to transfer electrons from plastoquinone directly to the oxygen and, thus, avoid a reduction in the plastoquinone pool, thereby protecting the chloroplasts from over-reduction (Laureau et al., 2013 ). Moreover, PTOX can keep the plastoquinol pool oxidized under cold, heat, or high-light stresses, and thus alleviate photoinhibition of PSII.
Here, we review the impact of major abiotic stresses, such as drought, heat, salinity, and heavy metals, on photosynthetic machinery, especially in agricultural crops, and the role of different phytohormones, transcription factors, and key enzymes involved in photosynthetic reaction center under stress conditions. Additionally, we highlight the utility of beneficial/stimulant compounds that help to maintain the viability and activity of the photosynthetic system. Lastly, we discussed some future perspectives that can improve photosynthesis under stressful conditions. We believe that this review provides a comprehensive summary of the photosynthesis related research conducted to date and will be useful for crop improvement in the future.
Major Factors Limiting Photosynthesis and Plant Growth
Effects of high temperature on the efficiency of photosynthesis.
Global increase in temperature is currently one of the biggest problems affecting plant survival. Rise in temperature above a certain threshold level impairs cellular homeostasis and plant metabolism, decrease in plant growth, biomass, and final yield components (Dutta et al., 2009 ; Ashraf and Harris, 2013 ; Mathur et al., 2014 ; Ye et al., 2016 ; Sharma et al., 2020 ). Low or freezing temperature affects photosynthetic parameters such as stomatal conductance, carbon reduction cycle, transpiration rate, and thylakoid electron transport (TET) (Hou et al., 2016 ). Photosynthesis is highly sensitive to heat stress (Wang D. et al., 2010 ; Centritto et al., 2011 ), as high temperatures disrupt the thylakoid membrane and inhibit membrane-associated electron carriers and enzymes, thus decrease the rate of photosynthesis (Ristic et al., 2008 ; Rexroth et al., 2011 ). Although plants are capable of fine-tuning their photosynthetic ability in response to high temperatures, but short-term extreme temperatures disrupt Chl biosynthesis within plastids, leading to reduced Chl accumulation, while high temperature for a longer time trigger the process of Chl degradation and even can cause irreversible damages to Chl synthesis (Ristic et al., 2008 ; Efeoglu and Terzioglu, 2009 ; Balouchi, 2010 ; Reda and Mandoura, 2011 ; Rexroth et al., 2011 ; Antoniou et al., 2017 ). Additionally, the photosynthetic apparatus senses the heat stress and responds by diverting the cellular energy to the redox center (Biswal et al., 2011 ). The photosynthetic apparatus is very sensitive to heat stress, as where the site of inhibition even more quickly responds than cellular disruptions (Mathur et al., 2014 ). Rubisco is the key photosynthesis enzyme and the enzymatic activity of Rubisco swiftly decreases by thermal stress which thereafter affect the process of photosynthesis (Anjana and Allakhverdiev, 2017 ). In several species, Rubisco activase (RCA) protein with molecular masses of 41 kDa (β-isoform) and 47 kDa (α-isoform) are capable of activating Rubisco (Salvucci et al., 1987 ), but they also have physiological significance in thermal sensitivity under heat stress conditions (Crafts-Brandner et al., 1997 ; Crafts-Brandner and Salvucci, 2004 ). Previously, it has been reported in rice (Wang D. et al., 2010 ) and spinach (Crafts-Brandner et al., 1997 ; Kim and Portis, 2006 ) that α-isoform is more thermostable than the β-isoform, indicating that the α-RCA isoform have crucial role in photosynthetic acclimation under mild heat stress ( in vivo ), whereas the β-RCA isoform have shown significant role in maintaining Rubisco's initial activity during normal condition. Thus, the genetic basis of RCA gene regulation and expression of two isoforms may be helpful for understand the mechanism of optimization Rubisco activation under prevailing environmental conditions
Noticeably, high temperature also disrupts the water-oxidizing complex (WOC) and the structural and functional integrity of the PSII reaction center and light-harvesting complex (LHC) (Lípová et al., 2010 ).
In plants, it has been reported that the high temperature inhibits Chl biosynthesis by decreasing the activity of biosynthetic enzymes (Dutta et al., 2009 ; Reda and Mandoura, 2011 ). For example, under heat stress the celery ( Apium graveolens L.) leaves, Chl biosynthesis declined because of the down-regulation of genes involved in Chl biosynthesis (Huang et al., 2017 ). Similarly, in barley ( Hordeum vulgare L.) seedlings, Chl biosynthesis was inhibited by high temperature treatment for 4–8 h; this was probably the result of the activity of 5-aminolevulinate dehydratase (ALAD), an enzyme actively involved in the pyrrole biosynthetic pathway, or the inhibition of protochlorophyllide (Pchlide) biosynthesis (Mathur et al., 2014 ). Previously, similar findings were reported in wheat ( Triticum aestivum L. cv. HD2329) seedlings, where the analogous effect of Pchlide biosynthesis resulted in identical outcomes (Tewari and Tripathy, 1998 , 1999 ). Furthermore, in soybean ( Glycine max L.), treatment with shift to high temperature (28–38°C) decreased the total Chl and Chl a contents by 18 and 7%, respectively, and the Chl a/Chl b ratio by 3% as well as the sucrose, sugar and leaf soluble sugar contents by 9, 47, and 36%, respectively (Tewari and Tripathy, 1998 , 1999 ; Mohanty et al., 2006 ). In young seedlings of cucumber ( Cucumis sativus L. cv. Poinsette), low (7°C) and high (42°C) temperatures caused irreversible damage to the photosynthetic apparatus, thus inhibiting plant growth (Tewari and Tripathy, 1998 ). In potato ( Solanum tuberosum L.), the thylakoid membrane was stable under moderately high temperature (35–45°C); however, a slight thermal stress (35°C for 2 h) decreased the electron transport and damaged the permeability of the thylakoid membrane (Sharkey, 2005 ), suggesting that de-epoxidized xanthophylls and thylakoid membranes are inefficient against heat-induced stress.
High temperature stress is usually accompanied by light stress, and the spatiotemporal cycles of both stresses damage the photosynthetic machinery (Tikkanen et al., 2012 ). The process of photoinhibition is associated with the thermosensitivity of PSII to high temperature. Mainly two key factors affect electron transport: (1) increased permeability of thylakoid membranes at high temperature, which results damage to the efficiency of PSII and the LHC, and (2) dependency of PSII integrity on electron transport (Janka et al., 2013 ). A previous study showed that moderately high temperatures with low light intensity do not cause serious damage to PSII but obstruct the repair of PSII after stress (Evans, 2013 ). PSI is more stable than PSII. Moderate heat stress stimulates PSI activity, characterized by the increased thylakoid proton conductance and electron flow around the PSI, by producing higher ATP. Subsequently, high NADPH/ATP ratio reduces the plastoquinone (PQ) pool in stromal donors, which activates the NADH-mediated cyclic electron flow (Sharkey, 2005 ; Sharkey and Zhang, 2010 ).
In spinach ( Spinacia oleracea L.), the intrinsic proteins of PSII cleaved the C-terminal end of the D1 protein under heat stress (40°C for 30 min), producing 9- and 23-kDa N-terminal fragments in thylakoids, and the slow repair process of the damaged D1 proteins reduced the cyclic electron flow under low light intensity, which negatively impacted plant growth and productivity (Yoshioka et al., 2006 ). Additionally, the FtsH protease, which localizes to the thylakoid stroma, translocated to the thylakoid granule to phosphorylate the D1 protein (Komayama et al., 2007 ). Here it can be assumed that D1 protein, degraded by heat and light stress (Nath et al., 2013 ).
Furthermore, the damage caused by heat stress in plants is attributed to the oxygen evolving complex (OEC), with alliance of cofactors in PSII and CO 2 fixation by Rubisco, which mostly affects the potential of yield, additionally, some research identified that the functionless Rubisco decreases net photosynthesis under slight temperature stress (Sharkey, 2005 ; Velikova et al., 2012 ; Yamori et al., 2014 ). In very early studies, it was shown that Rubisco catalyzes two contending pathways (photosynthetic CO 2 assimilation and photo-respiration), which are connected by the rate of oxygenase activity of Rubisco (Laing et al., 1974 ). Jordan and Ogren ( 1984 ) stated that the rate of photorespiration increases under high temperature, because of the relative specificity and relative solubility of CO 2 compared with that of O 2 (Jordan and Ogren, 1984 ). It has been reported that the lower expression of Rubisco per unit area under heat stress reduces the protein content in several species (Pérez et al., 2011 ). Although, plants grown in the natural environment generally exhibit great potential to tolerate high temperature, depending on the species, because of their adaptability and strong defense system. Figure 1 depicts the effect of heat stress on photosynthesis inhibition.

Schematic representation of the photosynthesis performance under abiotic stresses (heat, drought, and salinity). Drought and heat stress down-regulate enzymatic activity and electron transport chain (ETC) and cause membrane rupture, low CO 2 solubility, leaf senescence, and reactive oxygen species (ROS) production. On the other hand, salinity causes ion toxicity, membrane disruption, reduced stomatal conductance, lower quantum yield of PSII, slow electron transport, and reduced activity of photosynthesis related enzymes.
Moreover, an increase in the photosynthetic electron flux to oxygen (O 2 ) may lead to excess production of superoxide radicals, hydrogen peroxide (H 2 O 2 ) and other ROS that can damage proteins, lipids, and pigments (Li H. et al., 2017 ). Under low heat stress, ROS mainly disrupt the PSII repair system; however, it does not directly affect the PSII reaction center. Furthermore, the accumulation of compatible solutes, such as glycine betaine, in the vicinity of PSII membrane induces the expression of stress related proteins, and help in the stress damaged photosynthetic machinery (Evans, 2013 ). Thermal stress also induces changes in the total Chl and carotenoid contents of leaves, thus affecting the photoinhibition/photochemical intensity, resulting in reduced quantum yield of PSII (Fv/Fm), and enhanced peroxidation in the leaf cell membrane and decreased membrane thermostability, alter malondialdehyde (MDA) content, and electrolyte leakage (EL) (Cui et al., 2017 ; Zuo et al., 2017 ). These findings suggest that photosynthesis is sensitive to variation in temperature, and heat stress significantly affects the photosynthetic machinery, chlorophyll pigments, biosynthetic pathways, thus disturbing the overall morpho-physiology of higher plants. Identification of germplasm and development of transgenic lines with superior heat tolerance can be helpful to deal with high temperature stress.
Salt Stress Markedly Affects Photosynthesis
Excess salt or saline soil substantially alters biochemical and physiological processes, especially during photosynthesis, causing stunted plant growth, and poor productivity. Salt stress accounts for ~50% reduction in crop productivity (Gururani et al., 2015 ; Ahmad et al., 2018 ; Sharma et al., 2020 ). Moreover, salinity-induced osmotic stress reduces photosynthesis via the ionic effect on the structure of subcellular organelles and the inhibition of metabolic processes (Lawlor, 2009 ; Sade et al., 2010 ; Ahmad et al., 2020 ). The cellular membranes exhibit stress responses (Ashraf and Ali, 2008 ; Tayefi-Nasrabadi et al., 2011 ), high concentration of ions, such as sodium (Na + ) and chloride (Cl − ) ions, in chloroplasts causes significant damage to the thylakoid membrane (Wu and Zou, 2009 ; Omoto et al., 2010 ). Furthermore, inorganic salts at high concentrations can cause irrecoverable inactivation of photophosphorylation and obstruction of electron transport in the thylakoid membrane (Veiga et al., 2007 ; Mittal et al., 2012 ).
Previously, several studies showed that severe salt stress breaks down Chl, and the excess sodium ions Na + effect electron transport and destabilize photosynthetic activity (Pinheiro et al., 2008 ; Li et al., 2010 ). A reduction in photosynthetic pigments under salt stress was reported in several plant species such as wheat (Arfan et al., 2007 ; Perveen et al., 2010 ), alfalfa ( Medicago sativa ) (Winicov and Seemann, 1990 ), castor bean ( Ricinus communis ) (Pinheiro et al., 2008 ), and sunflower ( Helianthus annuus ) (Ashraf and Sultana, 2000 ; Akram M. S. and Ashraf, 2011 ). Najafpour et al. ( 2015 ) reported that the high Na + ion concentration in cells alters the potassium ion (K + ): Na + ratio, which instantaneously affects the bioenergetic processes of photosynthesis (degradation of photosynthetic pigments) in cyanobacteria as well as in plants (Najafpour et al., 2015 ). Similarly, Eckardt ( 2009 ) showed that salt-induced alterations impair the biosynthesis and accelerate the degradation of photosynthetic pigments (Eckardt, 2009 ). Other studies further summarized the reduction of Chl a and Chl b contents under salt stress in field crops, such as Paspalum vaginatum (Ivanov and Velitchkova, 2014 ), Centaurium erythraea (Sundby and Andersson, 1985 ), common bean ( Phaseolus vulgaris ) (Sundby and Andersson, 1985 ), Catharanthus roseus , cowpea ( Vigna unguiculata) (Taffouo et al., 2010 ), and Vigna subterranean (Muranaka et al., 2002 ).
Additionally, under salt stress, the Chl precursors, glutamate, and 5-aminolaevulinic acid (ALA), remarkably affect the biosynthesis of Chl in sunflower callus and plants (Vieira Santos et al., 2001 ; Santos, 2004 ). Salt tolerance plant species show an increase in Chl content, when grown under saline conditions (Khan et al., 2009 ; Akram N. A. and Ashraf, 2011 ), This lead to the concept that salt tolerant plant species with high Chl content exhibit greater membrane stability and higher Chl pigment content. So far, several salt tolerant plant species such as pea ( Pisum sativum ) (Noreen et al., 2010 ), melon ( Cucumis melo ) (Romero et al., 1997 ), sunflower (Akram N. A. and Ashraf, 2011 ), wheat (Raza et al., 2006 ; Arfan et al., 2007 ), alfalfa (Monirifar and Barghi, 2009 ), and proso millet ( Panicum miliaceum ) (Sabir et al., 2009 ) have been screened for their salt tolerance capacity. In contradiction to the aforementioned salt screening strategy, Juan et al. ( 2005 ) observed weak linkage between leaf Na + level and photosynthetic pigment content in tomato ( Solanum lycopersicum ) plants, indicating that chlorophyll content assimilation is not always associated with salt tolerance, but is an indicator of saline conditions, depending on the plant species (Juan et al., 2005 ).
A recent study revealed that salt stress (7–8 dS m −1 ) is also responsible for the reduction in the amount of carotenoids and Chl in sugarcane ( Saccharum officinarum L.) plants at different growth stages (Gomathi and Rakkiyapan, 2011 ). Another study in hot pepper ( Capsicum annuum L.) showed a significant increase in Chl and carotenoid contents in the presence of 60 mM salt (Ziaf et al., 2009 ). Therefore, we speculate that the carotenoid content of plants under salt stress could be a useful selection criterion. Additionally, salt tolerance at gene level has great potential; for example, the rice ( Oryza sativa L.) OsSUV3 gene, which encodes the Ski2 family of DExH/D-box helicases, functions under salt stress to facilitate photosynthetic processes and assist the antioxidant machinery (Tutej et al., 2014 ). Together, the studies described above prove that Chl content, photosynthetic pigments, membrane damage, and biochemical changes are of the primary targets under salt stress, where membrane instability and pigment degradation severely affect the growth, development, and physiological parameters of plants ( Figure 1 ).
Regulation of the Photosynthetic Machinery Under Drought Stress
Drought stress is one of the most crucial environmental factor impairing photosynthesis and thereby limiting plant growth and yield (Donohue et al., 2013 ; Hui et al., 2018 ; Tanveer et al., 2019 ). Water deficiency limits the efficacy of the photosynthetic apparatus, causes substantial damage to the thylakoid membrane and reduces the Chl content (Din et al., 2011 ; Smolikova et al., 2017 ; Demmig-Adams et al., 2018 ). Figure 1 links the photosynthesis-limiting drought stress with other abiotic stresses in the flow chart. Global warming and lower availability of underground water promote the occurrence of drought worldwide, thus affecting plant growth and productivity. To ensure survival under such a harsh environment, plants recruit their defense system and ultimately adjust themselves by adopting different strategies, such as stomata closure (to decrease transpiration), osmotic adjustment, and enhanced tolerance level (Zhang, 2007 ; Sharma et al., 2019 ). Xerophytes are an excellent example of such plants. Under drought stress, xerophytes absorb more water, reduce the transpiration rate, and exhibit morphological changes, such as a thick cuticle layer and stomatal closure (Macková et al., 2013 ). Beside that some non-stomatal mechanisms also decrease photosynthesis (Hajiboland et al., 2017 ); for example, the intake of CO 2 is disturbed by stomatal closure, which alters enzymatic activities, causes membrane disruption and reduces ATP synthesis and ribulose-1,5-bisphophate (RuBP) regeneration, thus inhibiting RUBISCO activity and effect the process of photosynthesis. Additionally, mild drought stress usually inhibits photosynthesis and stomatal conductance (Medrano et al., 2002 ), usually plants in such situation adopt defensive strategy by increasing the water use efficiency (WUE) by controlling net CO 2 and transpiration rate in leaf tissues (Chaves et al., 2009 ). By contrast, under severe drought stress, dehydration of mesophyll cells allows the utilization of available CO 2 , which remarkably inhibits the metabolic processes of photosynthesis, leading to reduction in WUE and root hydraulic conductivity (Karaba et al., 2007 ; Dias and Brüggemann, 2010 ; Anjum et al., 2011 ; Damayanthi et al., 2011 ; Din et al., 2011 ).
Additionally, drought exerts a negative effect on the PSII by reducing its quantum yield (Albert et al., 2011 ; Tattini et al., 2014 ). A study disclosed that during drought stress, the turgor pressure of cells decreased, which reduced the shoot length, biomass, and plant growth (Semerci et al., 2017 ). The photosynthetic machinery is significantly affected by water-deficit condition (Sun et al., 2013 ); for example, water deficiency results in the degradation of the thylakoid membrane and Chl pigments and decreases the Chl content (Bertioli et al., 2016 ). Moreover, altered Chl fluorescence kinetics affects PSII (Zhang et al., 2011 ). This was confirmed by Batra et al. ( 2014 ); where the reduced Chl fluorescence and water content, creates dehydration, and further targeting PSII electronic transport and PQ reduction under water-deficit condition (Batra et al., 2014 ).
Teixeira et al. ( 2016 ) reported that the Chl content of soybean seeds under transient drought stress had showed effects on nutritional value and oil quality (Teixeira et al., 2016 ). Additionally, the impaired expression of SGR (STAY-GREEN), a chloroplast targeted protein that act as key regulator of Chl degradation and NYC1 (NON-YELLOW COLORING 1) under heat and drought stress inhibit the process of chlorophyll degradation and retention in green soybean seeds. In wheat, the relative contribution of ear (spike and awns) to grain filling is severely affected by drought stress, for instance the CO 2 assimilation is reduced due drought and eventually disturb the rate of photosynthesis (Kottmann et al., 2014 ; Merah et al., 2018 ). Ashraf and Harris ( 2013 ) reported that Chl content assimilation does not show a positive correlation with drought condition in wheat plants, suggesting that it may be due to variation in Chl synthesis among the cultivars mediated by the alteration in the activities of specific enzymes involved in the biosynthesis of Chl (Ashraf and Harris, 2013 ). Despite the above contradiction in the assimilation of Chl content, degradation, and biosynthesis under drought stress has been reported by various researchers; for example, in some genotypes of black gram ( Vigna mungo ), Hamada and Al-Hakimi ( 2001 ) and Pirzad et al. ( 2011 ) reported an imbalance in accumulation of Chl (Hamada and Al-Hakimi, 2001 ; Pirzad et al., 2011 ). However, the regulation of enzymatic activity during Chl biosynthesis is dependent on the response of a particular genotype. Additionally, enzymatic activity of chlorophyllase and peroxidase is involved in the rapid breakdown of Chl that reduce the process of synthesis (Kaewsuksaeng, 2011 ). Moreover, a greater amount of Chl b than that of Chl a under drought condition has been reported by Jaleel et al. ( 2009 ) and Jain et al. ( 2010 ). In Brassica and wheat, the Chl a/b ratio was highly reduced in susceptible cultivars under drought stress, but was slightly increased in tolerant genotypes (Ashraf and Mehmood, 1990 ; Ashraf, 1994 ). In rice, helicase domain-containing proteins have shown up-regulation in response to drought stress and functions as maintaining photosynthesis and antioxidant machinery (Ambavaram et al., 2014 ; Chintakovid et al., 2017 ), Moreover, the genes encoding GAPDH and FNR [the key enzymes influencing NADP(H) homeostasis are affected by osmotic-stress treatments], suggests that drought tolerance in rice may be mediated by photosynthesis-related adaptations by utilizing the NADP(H) homeostasis.
Effect of Heavy Metal Pollution on the Photosynthetic Machinery
Soil contamination with heavy metals such as cadmium (Cd), copper (Cu), zinc (Zn), nickel (Ni), cobalt (Co), chromium (Cr), lead (Pb), and arsenic (As) is generally caused by the application of phosphate-rich fertilizers, sewage sludge, industrial waste, wind-blown dust, incinerator emissions, traffic, volcanoes, and hard water practices (Bagur et al., 2009 ; Ghori et al., 2019 ). Heavy metal pollution considerably inhibits plant growth by causing Chl degradation, DNA and protein damage as well as enzymatic inhibition ( Figure 2 ). When coupled with other environmental stresses, heavy metal pollution causes more severe damage (Srivastava et al., 2012 ; Kumar et al., 2019 ; Sharma et al., 2020 ). Abiotic stresses like heavy metals induce excessive accumulation of ROS and cause oxidative stress in plants (Li et al., 2016 ). Interestingly, metallothioneins (MTs) have been proposed an alternative tool by which plants protect themselves from stress-induced oxidative damage (Yu et al., 2019 ). Hassinen et al. ( 2011 ) reported the role of MTs in abiotic stress tolerance as ROS scavengers, though the mechanisms through which MTs mediate ROS homeostasis remain unclear (Hassinen et al., 2011 ).

Diagram showing the general and specific effects of heavy metals on plants. Heavy metals affect ROS production, inhibit ATP synthesis and damage DNA and proteins. The damage to DNA and proteins is shown separately. Free radicals damage DNA and cause membrane peroxidation when metal ions surround the site of tissue injury, causing ATP inhibition, and NADH depletion.
Moreover, heavy metal stress considerably alters the biological, biochemical and metabolic processes of plants (Anjum et al., 2015 , 2016a , b , c ; Handa et al., 2018 ; Shahzad et al., 2018a ; Khanna et al., 2019 ; Kohli et al., 2020 ), and alteration at the cellular and molecular levels causes severe damage, thus blocking the functional group and active site of enzymes, thereby disrupting membrane stability and transcriptional regulation (Rascio and Navari-Izzo, 2011 ; Guo et al., 2016 , 2017b ). Metal ion toxicity causes DNA damage and/or impairs DNA repair mechanisms, disrupts membrane functional integrity, affects enzymatic activity, and perturbs protein function (Tamás et al., 2014 ; Figure 2 ).
LHCII (light harvesting complex II) is the basic pigment-protein complex of PSII, which harvests light energy and converts it into chemical energy. This protein complex plays a protective role by dissipating excess light energy and efficiently channelize excitation energy (Barros et al., 2009 ). Cd stress affects the LHCII (Parmar et al., 2013 ). In rye ( Secale cereale ), Cd stress reduced the dissipation of excitation energy, indicating that Cd stress either altered the quenching center (QC) or interfered with energy transfer between proteins and pigments (Janik et al., 2010 ). Ahmed and Tajmir-Riahi ( 1993 ) confirmed changes in LHCII by Pb, where imperfect assembly of its components triggered disintegration (Ahmed and Tajmir-Riahi, 1993 ).
Plants grown in metal contaminated soils exhibit leaf chlorosis due to the reduced chloroplast size (Shahzad et al., 2016 , 2017 ). The ultra-structure of chloroplast is greatly affected by metal ions ( Figure 3 ). For example, Cd, a potent inhibitor of photosynthesis, alters the chloroplast shape, decreases chloroplast size, destroys Chl, reduces starch accumulation and expands the thylakoids (Najeeb et al., 2011 ; Parmar et al., 2013 ; Kapoor et al., 2019 ). Other metals such as Cr decrease the absorption of magnesium (Mg) and nitrogen (N), consequently reducing the Chl content (Singh et al., 2013 ). Pb toxicity accelerates Chl degradation by increasing the activity of chlorophyllase (Drazkiewicz, 1994 ). High levels of Ni affect the photosynthetic apparatus and inhibit the synthesis of pigments (Soares et al., 2016a , 2019 ; Shahzad et al., 2018a , b ). Additionally, Ni stress alter the composition of lipid membrane and disturb the activity of chlorophyll molecule and Rubisco (ribulose-1,5-bisphosphate carboxylase oxygenase) (Kohli et al., 2020 ).

Heavy metal stress damages the chloroplast structure and chlorophyll (Chl) biosynthesis and degradation processes during photosynthesis. Toxicity due to metal ions gradually affects enzymatic activity and inhibits Chl components and the uptake of essential elements, finally blocking the Chl biosynthesis pathway. The blue arrow depicts this process in a step-by-step manner.
Early studies established that the photosynthetic apparatus, Chl and carotenoid concentrations and plant growth were greatly decreased under Ni and UV-B combination stress. It is possible that Mg in Chl is replaced by Ni, which destroys Chl and damages thylakoid membranes in cabbage leaves and wheat shoots, respectively (Molas, 2002 ; Gajewska et al., 2006 ). Similarly, the combined effect of heavy metals and drought stress can cause deleterious effects on Chl content in red maple and effect the xylem structure and hydraulic conductivity (De Silva et al., 2012 ).
Transition metals (Cu, Zn, manganese [Mn], and iron [Fe]) play critical roles in physiological processes of all living organisms, such as Cu is essential for respiration, photosynthesis, cell wall integrity, ethylene perception, and ROS metabolism in plants (Burkhead et al., 2009 ). Excess amounts of transition metals leads of Chl degradation by Chl-degrading enzymes, thereby increasing the sensitivity of PSII to light (Pätsikkä et al., 2002 ). Cu, Hg, Ni, Zn, and aluminum (Al) metal ions interact with three extrinsic polypeptides, situated in the lumen of the thylakoid membrane, additionally, intrinsic proteins (inner antenna protein), are released from the PSII reaction center under Cu toxicity (Sigfridsson et al., 2004 ; Boisvert et al., 2007 ). At optimum concentrations, Zn plays an important role in plant growth; however, excess Zn significantly reduces the synthesis of photosynthetic pigments and negatively impacts photosynthesis (Paunov et al., 2018 ). Zn and Cd stresses exert a synergistic effect by increasing the oxidative stress and restoring the Chl content (Cherif et al., 2011 ). Therefore, we speculate that transition metals in combination may reduce or restore the damage caused to the photosynthetic machinery for a short period of time under specific conditions. Comparative analysis of Cd and Zn stresses in tobacco ( Nicotiana benthamiana ) leaves revealed that under Cd stress, key enzymes involved in Chl biosynthesis were significantly down-regulated, decreasing the Chl content, expression of PSII (donor, receptor and core side) proteins and photosynthesis (Zhang et al., 2020 ). Several studies have reported the harmful effects of Cd and Zn on photosynthesis, thylakoid membrane ultra-structure, photosynthetic pigments, Chl fluorescence, electron transport, light capture, dark respiration, stomatal conductance, and Calvin cycle enzymes (Krupa, 1999 ; Vassilev et al., 2011 ; Paunov et al., 2018 ). Furthermore, both metals considerably reduce the activity of PSII and to some extent that of PSI as well as the rate of photosynthetic electron transport (Krupa, 1999 ; Vassilev et al., 2004 ).
Impact of Light Intensity on Photosynthesis
Light is the key factor responsible for the healthy growth and development of plants, and fluctuation in light intensity negatively affect plant physiology and biochemistry and inhibit the process of photosynthesis (Demmig-Adams and Adams, 1992 ; Berenschot and Quecini, 2014 ; Wang Y. et al., 2017 ; Sharma et al., 2020 ). For instance, high light intensity generates harmful oxygen radicals (Gururani et al., 2015 ) and disrupt LHC (light harvesting complex), thus causing photoinhibition (Erickson et al., 2015 ), whereas insufficient light reduces the photosynthetic efficiency as well as CO 2 and N metabolism and boosts oxidative stress (Solymosi and Schoefs, 2010 ; Wang et al., 2013 ). Additionally, low light significantly reduces photosynthesis and the efficiency of stomatal conductance, resulting in a rapid increase in the intercellular CO 2 concentration in leaves (Liu et al., 2014 ). Thus, low light stress inhibits the photosynthetic machinery, stomatal conductance, maximum quantum efficiency of PSII, transpiration rate, WUE, and net photosynthesis rate (Pan and Guo, 2016 ; Zhang et al., 2016 ). Previous reports described that photosynthetic organisms are frequently exposed to high light intensities, in order to avoid photo-oxidative stress, plants adopt a photo protective mechanism by inducing non-photochemical quenching (NPQ) for safe dissipation of the excess energy as heat (Demmig-Adams and Adams, 1992 ; Erickson et al., 2015 ; Tibiletti et al., 2016 ).
Phytochromes and cryptochromes are multiple sensory photoreceptors that respond to light signals. However, the mechanistic explanation of these phytochromes is not yet fully understood. The function of direct protein–protein interaction, SPA1/COP1 E3, ubiquitin ligase complex, bHLH transcription factors, and transcription regulation of BIC genes regulates the photoreceptor coactions, which may serve as a safe valve to prevent cryptochromes from over-reacting in germinating seedlings (Wang Y. et al., 2017 ; Wang et al., 2018 ). Photoinhibition is caused by the functional failure of the PSII reaction center (Gururani et al., 2015 ), where poor oxidation during high light intensity damages the intrinsic key responsible D1 protein (Chen et al., 2012 ) and degradation in PSII reaction center become photo-inactivated. Additionally, high light intensity also reduces mitochondrial activity, photochemical efficiency as well as the dissipation of excess light energy in the form of heat, thus damaging PSI and PSII (Faseela and Puthur, 2018 ). Additionally, the quantum efficiency of PSII is hindered by electron transport, photochemical efficacy, photo-oxidation under high light stress accompanied by high temperature (Faseela and Puthur, 2017 ). Furthermore, high light intensity induces Chl b degradation by the Chl b reductase enzyme (Sato et al., 2015 ), although plants have the ability to modify their physiology by altering processes such as stomatal conductance and transpiration (Cowie et al., 2016 ).
Severe light stress also causes photodamage in the photosynthetic apparatus. For example, in tropical regions, plants face high light intensity with high temperature, which causes photon excitation in the chloroplast and reduction of photochemical efficiency (Elsheery and Cao, 2008 ). On the other hand, leaves of plants grown in shade absorb excess light energy, leading to photoinhibition (Krause et al., 2004 ; Takahashi et al., 2009 ). Additionally, the efficiency of PSII also decreases when the upper leaves are growing in shade, although the performance of PSI is not significantly affected under these conditions (Barth et al., 2001 ; Krause et al., 2004 ). The sensitivity of PSI to photoinhibition was also observed in Arabidopsis thaliana and cucumber during chilling (Zhang and Scheller, 2004 ). Xu et al. ( 2016b ) observed that high light intensity (1,000 μmol photons m −2 s −1 ) causes photodamage in the cells of Dunaliella salina , while free hydroxyl radicals induce PSI photoinhibition via oxidation (Sonoike, 2011 ; Xu et al., 2016b ).
ROS production during photosynthesis is regulated by the interaction of O 2 with the photosynthetic electron transport chain (ETC) (Li et al., 2009 ). Under excess light, ROS production increases, and to avoid the accumulation of ROS to harmful levels, plants employ antioxidant enzymes, detoxification, and repair mechanisms (Falk and Munné-Bosch, 2010 ; Pospíšil, 2014 ). Additionally, the production of ROS by high light intensity induces plant cell death (González-Pérez et al., 2011 ). Plastoquinone-9, a prenyl lipid that serves as an electron carrier between PSII and PSI, protects the photosynthetic apparatus by controlling photoinhibition of PSII under severe light stress (Ksas et al., 2015 ).
Effect of Phytohormone Signaling Networks on Photosynthesis Under Stress
The interaction of plant hormones and cellular redox is crucial for the process of photosynthesis under different abiotic stresses (Mayzlish-Gati et al., 2010 ; Kim et al., 2012 ; Krumova et al., 2013 ). Previously, it was evident that impaired photosynthetic light harvesting in Arabidopsis mutants has strong interaction between the control of excitation energy transfer and hormonal regulation (Tikkanen et al., 2014 ). The metabolism of phytohormone regulation network by ROS generation may intricate to complex hormonal crosstalk in response to stressful conditions presented in Table 1 .
Effects of plant hormones on the photosynthetic machinery under normal and stress conditions in different plant species.
Strigolactones (SLs) play an important role in the regulation of genes associated with harvesting light. Synthetic SL compounds regulate several genes encoding LHC proteins, CAB proteins, and PSI and PSII components under stress conditions (Mayzlish-Gati et al., 2010 ). For example, in the Arabidopsis SL signaling mutant max2 , the response to dehydration is down-regulated because of the suppression of genes involved in photosynthesis, suggesting that the association between the misregulation of genes involved in photosynthesis reduced drought tolerance and sensitivity to the high energy demands of photosynthesis in max2 plants (Ha et al., 2014 ).
Gibberellins (GAs) regulates photosynthesis and promote seed germination and cell division (Huerta et al., 2008 ; Zhou et al., 2011 ). In cucumber cotyledons, GA and kinetin influence the functional site of PSI and PSII reaction centers, thereby encouraging the development of the photosynthetic electron transport system (Pedhadiya et al., 1987 ). Similarly, in broad bean protoplasts, short-term GA-3 treatment increased the net photosynthetic rate and O 2 evolution (Yuan and Xu, 2001 ). In transgenic Brassica napus plants, the photosynthetic capacity increased with the decrease in GA bioactivity (Zhou et al., 2011 ). Higher levels of endogenous GA remarkably up-regulate genes involved in photosynthesis and drought tolerance (Huerta et al., 2008 ). In wild-type and transgenic Arabidopsis plants, GA-3 treatment activated GA-responsive genes and enhanced tolerance to heat, salt and oxidative stresses (Alonso-Ramírez et al., 2009 ). However, further studies are required to exploit the relationship between photosynthesis and endogenous/exogenous GA-3 levels under various abiotic stresses.
Brassinosteroids (BRs) play important roles in plant growth and development, abiotic stress responses and defense mechanism. BRs also influence the efficiency of PSII and photosynthetic CO 2 fixation in land plants (Oh et al., 2010 ; Choudhary et al., 2012 ; Krumova et al., 2013 ). Previous reports uncovered the relationship between BRs and photosynthesis related genes in several plant species (Oh et al., 2011 ; Bai et al., 2012 ). For instance, in the Arabidopsis brassinosteroid-insensitive1 ( bri1 ) mutant, genes involved in the regulation of photosynthesis were significantly down-regulated, which reduced plant growth and photosynthetic activity (Kim et al., 2012 ). Moreover, further analysis revealed that Arabidopsis mutants with altered BR responses exhibit drastic changes in thylakoids, inhibition of O 2 evolution, reduction in PSII quantum yield and smaller PSII complex (Krumova et al., 2013 ). The BR-induced changes in the thylakoid structure and regulation of PSII during photosynthesis have also been described in other studies (Dobrikova et al., 2014 ; Rothová et al., 2014 ). Although BR deficiency boosts the content of Chl and photosynthetic proteins in plants, changing the leaf color to dark green (Komatsu et al., 2010 ), exogenous BR treatment in pepper ( Capsicum annuum ) resulted in harmful effects on photosynthesis under drought stress by decreasing light use efficiency and non-photochemical quenching (NPQ) in PSII antennae (Hu et al., 2013 ). Based on the abovementioned studies, it can be concluded that intensive investigation should be practiced to describe the specific role of BRs in the PSII damage repair system and ameliorating changes in the thylakoid structure during the process of photosynthesis.
The most widely studied hormone, abscisic acid (ABA), plays a dynamic role in response in plants during abiotic stresses. ABA directly regulates the PSII-associated O 2 evolution and granular chloroplast structure in plants (Maslenkova et al., 1989 ). Exogenous supply of ABA enhanced the amount of Chl, total carotenoids, and xanthophylls in leaves, and also help in excessive excitation energy on PSII (Barickman et al., 2014 ). In barley seedlings, ABA treatment significantly increased the photosynthetic apparatus under heat stress, although heat shock reduced the damage to the initial chloroplast fluorescence (Ivanov et al., 1992 ). ABA treatment up-regulates the expression of LHCB gene family, which is mainly involved in the adaptation to abiotic stress (Liu et al., 2013 ). Additionally, down-regulation of LHCB genes decreased ABA signaling, suggesting the involvement of ABA signaling in drought stress and ROS modulation (Xu et al., 2012 ). The inconsistency concerning the role of ABA in photosynthesis is possibly due to the dissimilar experimental system/methodology and the photosynthesis data obtained using different methods.
Salicylic acid (SA), a phenolic compound, is extensively involved in the process of plant growth regulation, physiology and biochemical activities of cells as well as in the response to stress conditions (Kunihiro et al., 2011 ; Drzewiecka et al., 2012 ; Li Z. et al., 2017 ). Therefore, it is important to discover the role of SA related genes in photosynthesis during abiotic stresses. Investigation in Phillyrea angustifolia plants under drought stress showed increased assimilation of endogenous SA and significantly decreased Fv/Fm in leaves (Munné-Bosch and Peñuelas, 2003 ). SA treatment improved the photosynthetic capacity of wheat plants (Arfan et al., 2007 ). Similarly, SA treated wheat leaves showed an improvement in Fv/Fm, photochemical activity of PSII, photosynthetic rate and electron transport, which reduced the damage caused by heat and high light intensity to the D1 protein and PSII (Zhou et al., 2011 ). Therefore, it can be established that SA pretreatment is associated with the chloroplastic heat shock proteins (HSPs), thereby up-regulating the photosynthetic rate. In other crops such as barley, exogenous SA treatment facilitated the adaptation to salt stress and improved the cell membrane integrity (El-Tayeb, 2005 ). In grapevine ( Vitis vinifera ) leaves, SA treatment under heat stress enhanced the PSII system parameters and net photosynthetic rate (Wang L. J. et al., 2010 ).
To date, only a few studies have described the roles of methyl jasmonate (MeJA) and ethylene in photosynthesis, and the regulatory mechanisms that provide stability to PSI and PSII under abiotic stresses remain unclear. However, exogenous application of ethylene demonstrated its role in the regulation of tolerance to Ni- and Zn-induced heavy metal stress by improving photosynthetic efficiency (Khan and Khan, 2014 ). Additionally, in sunflower, ethylene treatment increased the net photosynthetic rate and reduced the effects of excess Cu, thereby stabilizing the Fv/Fm ratio (Ouzounidou and Ilias, 2005 ).
Selenium (Se) guards the photosynthetic activity of B. napus seedlings under Cd stress, as high Cd-stressed plants may act as a trap for free radicals stabilized by the starch matrix (Filek et al., 2010 ). Similarly, calcium (Ca) modification overturned the Cd stress-induced changes and increased the intercellular CO (2) concentration and NPQ as well as defied Cd accumulation in B. napus seedlings (Wan et al., 2011 ). In transgenic tobacco plants, cytokinin (CK) was shown to improve photosynthetic efficiency, cytochrome b6f (Cytb6f) complex formation, photosynthetic apparatus, and expression levels of genes associated with PSI and PSII as well as delay drought stress (Rivero et al., 2007 , 2010 ). Figure 4 displays the complex hormonal crosstalk and regulation of stress-associated factors under stressful conditions. Thus, the studies on phytohormones provide fundamental background information about their potential crosstalk under stress conditions, but gene regulation and plant response could be more easily explained after the mutational studies, which will further disclose the comprehensive approaches and specific roles of different plant hormones in photosynthesis under both normal and stress conditions.

Regulation of the complex phytohormone network under stress conditions. The imposition of stress on the photosystem reaction center induces the hormonal signal transduction. The red dotted lines indicate the regulation of two hormone inhibitions, and green lines indicate the co-regulation of two hormones. The regulation of gene transcription by different hormones shows the involvement of the photosynthetic machinery.
In addition to the analysis of individual phytohormones, some studies investigated the co-regulated hormonal responses to various stresses. For example, ABA and SLs together regulate carotenoid biosynthesis (Barickman et al., 2014 ), suggesting that the potential crosstalk between ABA and SLs (carotenoid-derived hormones) plays a role in the light-harvesting pathways. Recently, it was found that BR-dependent and GA-regulated transcriptome involved in cell wall formation and photosynthesis, the evidence indicates a strong association between various hormones and light-harvesting pathways (Attaran et al., 2014 ; Cortleven et al., 2014 ). Moreover, studies have reported that BRs modulate PSII efficiency and thylakoid architecture (Oh et al., 2011 ; Krumova et al., 2013 ; Dobrikova et al., 2014 ), which suggests that the coordinated crosstalk between BR and GA-signaling network regulates the adaptive response of plants to adverse environmental conditions.
Additionally, the coordination and crosstalk among phytohormone signaling networks somehow established the adaptive responses of plants under unfavorable conditions (Nishiyama et al., 2011 ; Ha et al., 2014 ). For example, abundance of ethylene during drought stress triggers leaf senescence and also disturbs ABA-mediated regulation of photosynthesis in leaf expansion (Bartoli et al., 2013 ). This suggests that the relation between ethylene and ABA and their relative abundance control the response behavior of plants to drought stress. Further studies are required to measure the effect of co-regulated hormones and points of intersection, which alter the regulation of the photosynthetic machinery or its components and reduce photoinhibition in chloroplasts under stress conditions. Moreover, we propose that additional genes and their roles in chloroplast should be identified to improve photosynthesis under abiotic stress.
Effects on Activities of Key Photosynthetic Enzymes
The photosynthetic efficiency swiftly changes by various enzymatic reactions within cells during abiotic stresses (Kumar and Singh, 2009 ; Gill et al., 2011 ). The utmost noticeable effect of many stresses is the stomatal conductance and closure, which reduces the intercellular CO 2 concentration by the deactivation of various enzymes such as Rubisco, sucrose-phosphate synthase (SPS), and nitrate reductase (Chaves et al., 2009 ; Mumm et al., 2011 ). Under high saline stress, the metabolic processes of photosynthesis are affected, and the thermostatic pressure is activated by a number of stroma enzymes that reduce CO 2 (Xue et al., 2008 ; Biswal et al., 2011 ). Additionally, the activity of Rubisco is inhibited both in vitro and in vivo under high salt stress (Aragao et al., 2005 ). In general, the enhanced activity of Rubisco provides stability to plants under stress conditions, and the leaf rubisco level shows a positive relationship with phosphorous (P) and N levels in most C 3 plants, except in glycophytic species; however, a contrasting relationship is detected under salt stress (Aragao et al., 2005 ; Taub, 2010 ; Makino, 2011 ). This contrasting equation needs further investigation to clarify the relationship between these traits. Ghosh et al. ( 2001 ) reported that under saline conditions in rice, two genotypes not only affect Rubisco but also its substrate, RuBP, which plays a key role in the Calvin cycle (Ghosh et al., 2001 ). Additionally, Pb affects CO 2 fixation by altering RuBP and phosphoenol pyruvate carboxylase (PEPC) in the C3 and C4 cycles, respectively (Agarie et al., 2002 ; Häusler et al., 2002 ). Moreover, Pb also disturbs the activity of glyceraldehyde-3-phosphate dehydrogenase (GAPDH) and ribulose-5-phosphate kinase in the C3 cycle. Drought-responsive ABA hormone is mainly regulated by 9-cis-epoxy carotenoid dioxygenase (NCED) and d-arabino-1,4-lactone oxidase (ALO), enabling plant resistance (Bao et al., 2016 ). This is because ABA controls the balance between stomatal conductance and transpiration rate to prevent water loss under drought stress (Pirasteh-Anosheh et al., 2016 ). Moreover, some studies revealed that the photosynthetic efficiency remarkably reduced by fructose-1,6-bisphosphatase during stress. A significant reduction in the RuBP pool size has been reported in common bean (von Caemmerer and Farquhar, 1984 ), sunflower (Gimenez et al., 1992 ), and rice (Ghosh et al., 2001 ).
The activity of phosphoenolpyruvate carboxylase (PEPC) in wheat (C 3 plant) and maize (C 4 plant) is inhibited by salt stress, although the PEPC of wheat is less sensitive to salt stress than that of maize. Hirel et al. ( 2007 ) described the potential utility of the Rubisco content (i.e., improved WUE and yield components) in breeding programs (Hirel et al., 2007 ). Maize transgenic plants with enhanced expression of C 4 -PEPC showed 30% increase in WUE and 20% increase in dry biomass under water scarcity, which suggests that the maize transgene approach can be used as a tool improving the endogenous enzymes involved in the process of photosynthesis (Jeanneau et al., 2002 ). Previously, it was reported that the activities of the various C 4 photosynthesis enzymes, such as PEPC, NADP-malic enzyme (NADP-ME), Rubisco and fructose-1,6-bisphosphatase were greatly limited under drought stress (Du et al., 1998 ). For example, the activity of pyruvate phosphate dikinase (PPDK) was reduced in C 4 plants, which confirmed the role of PPDK in photosynthesis under drought conditions. Li et al. ( 2007 ) reported a reduction in the solubility of CO 2 in the leaf tissue and regulation of the Rubisco enzyme at high temperatures, consequently reducing photosynthesis (Raines, 2011 ). Crafts-Brandner and Salvucci ( 2004 ) reported that Rubisco activity was significantly inhibited in both cotton and tobacco, when the leaf temperatures were exceeded at 35°C. A similar mechanism of the acclimation to high temperatures in C 4 maize plants had showed association with manifestation of a larger subunit of Rubisco and limited recovery of the Rubisco activation state (Crafts-Brandner and Salvucci, 2002 ). This indicates that the decrease in Rubisco activation is highly affected by increase in temperatures. Chinthapalli et al. ( 2003 ) reported that PEPCase from the C 4 plant (Amaranthus hypo-chondriacus) showed less sensitivity to supra-optimal temperatures and more sensitivity to sub-optimal temperatures as compared to the enzymes in C 3 species ( Pisum sativum ) (Chinthapalli et al., 2003 ). It might suggest that the key photosynthetic enzymes in both C 3 and C 4 plants shows variation in sensitivity to higher temperatures.
Although all C 4 plants exhibit greater tolerance to high temperature than C 3 plants, the photosynthetic efficiency of C 4 plants relatively sensitive to heat stress, probably due to the activation of Rubisco in C 4 plants, which inhibits electron transport and PEP carboxylation/PEPC activity and regeneration. In the light of these findings, it can be concluded that the key photosynthetic enzymes in C 3 and C 4 plants exhibit different levels of sensitivity to temperature. Our conclusion is justified by Xu et al. ( 2003 ), who showed temperature-sensitive activity of photosynthesis related enzymes; in wheat plants under heat stress, Rubisco activity was reduced after 12 days, and PEPC activity remained unstable while the ratio of PEPC/Rubisco markedly increased. Enzymatic activities also reshape the photosynthetic pathways in C 3 and C 4 plants under various abiotic stresses, depending on several factors such as stomatal response, plant type, and interaction with enzymatic changes, thus altering the photosynthetic capacity. When plants are encountered by abiotic stresses, GAPDH converts glycerate-3-phosphate (G3P) to glyceraldehyde-3-phosphate, and the latter further promotes the ability to receive electrons from NADPH and rescue PSII from ROS (Hildebrandt et al., 2015 ). Previously, it was observed that GAPDH is up-regulated in wheat genotypes by PEG6000 treatment for 48 h, indicating that GAPDH plays a vital role in the maintenance of photosynthetic activity and promotion of plant growth (Cheng et al., 2015 ). Additionally, overexpression of the GAPDH gene in transgenic tobacco plants enhanced tolerance to drought stress (Kappachery et al., 2015 ). Another enzyme, ferredoxin-NADP reductase (FNR), helps to channelize electronic transport and redox homeostasis within chloroplasts (Chinthapalli et al., 2003 ). Different abiotic stresses affect the activity of FNR differently; for example, in transgenic tobacco plants, FNR level was reduced by drought stress (Gharechahi et al., 2015 ), whereas in Paeonia cathayana (Xiao et al., 2009 ), wheat (Budak et al., 2013 ), rice (Nouri et al., 2015 ; Chintakovid et al., 2017 ), and maize, FNR levels was increased by salt stress (Zörb et al., 2009 ). In rice, GAPDH activity was up-regulated, whereas FNR level was reduced under osmotic stress (Chintakovid et al., 2017 ). These outcomes indicate that different plant species employ different mechanisms to stabilize the electron flow during photosynthesis. Additionally, the activation of numerous enzymes is concerned with light, therefore, the light intensity and duration of intervals is another stress, that decide the activation, or deactivation of these enzymes. Additionally, enzymes are generally very sensitive their environment, and even small environmental fluctuations can affect their potential stability and functionality.
Transcription Factors (TFs) and Their Association With Photosynthesis Under Stress Conditions
Gene expression is critically controlled by TFs that manipulate various cellular processes in almost all living organisms, although TF regulation for particular gene/genes is fundamentally dependent on the genome size of a given species. Hence, the main objective of researchers is to identify the molecular mechanism underlying gene expression and gain functional insights into the role of the specific gene/genes affecting specific traits control through regulation by TFs (Ashraf and Harris, 2013 ).
The function of various TFs involved in the regulation of photosynthesis related genes, either directly or indirectly (hormonal pathways), has been investigated previously (Saibo et al., 2009 ; Gururani et al., 2015 ). Plant TFs such as BZR1 and WRKY , have been noticed to influence cell-wall and genes related to chloroplast, additionally, the efficiency of PSII system have shown correlation with the regulation of GhDREB and CRF6, and chlorophyll content (Waters et al., 2009 ; Nguyen et al., 2014 ). The regulation of CAB gene expression by the light-responsive LONG HYPOCOTYL 5 (HY5) and bZIP-type TFs plays a critical role in stress tolerance (Saibo et al., 2009 ). The CAB2 TF not only controls the expression of Rubisco but also regulates the expression of RbcS1A (a gene that encodes subunit 1A of Rubisco) (Maxwell et al., 2003 ; Lee et al., 2007 ). Similarly, the rice OsMYB4 TF is responsible for the accumulation of glycine betaine, which increases the adaptability of Arabidopsis plants under stress conditions. Moreover, glycine betaine improves the structure of Rubisco under saline conditions (Yang et al., 2005 ; Khafagy et al., 2009 ). In maize, the two TFs including DOF1 (activator) and DOF2 (repressor) regulate the expression of the PEPC gene under stress conditions (Yanagisawa and Sheen, 1998 ; Yanagisawa, 2000 ). Another study unveiled that CAM (Calmodulin)-specific genes in plants show high expression levels because of cis -acting and trans-acting transcriptional regulation under drought and salt stress conditions (Saibo et al., 2009 ). The MYB-type, Gap encoding NAD-dependent GAPDH, and Ppcl gene encoding a CAM-specific isozyme of PEPC regulation induced under saline stress and further activates photosynthesis-related genes (Schaeffer et al., 1995 ). Constitutive expression of ABP9 and bZIP genes in transgenic Arabidopsis plants regulates pigment composition, photosynthetic carbon and ABA level in leaf tissue as well as activate after receiving stress signals (water deficit and heat stress) and involve in light harvesting conditions (Zhang et al., 2008 ). The C-repeat binding factor/dehydration-responsive element (CRT/DREB)-binding TF family controls the expression of key genes and sense environmental cues and actively coordinates signal transduction networks (Agarwal et al., 2017 ).
The expression of CBF/DREB genes respond to temperature fluctuations in Arabidopsis (Schramm et al., 2008 ; Demmig-Adams et al., 2018 ) as well as in other plant species (Akhtar et al., 2012 ; Kurepin et al., 2013 ; Kidokoro et al., 2015 ; Agarwal et al., 2017 ). The CBF/DREB TF family is divided into two groups; TFs in one group are primarily associated with cold stress adaptation, while those in the second group are predominantly involved in heat, drought, and salt adaptation (Demmig-Adams et al., 2018 ). The CBF1, CBF2 , and CBF3 genes, also known as DREB1B, C, and A, respectively, are dynamically involved in plant growth regulation under cold stress (Thomashow, 2010 ). DREB2 TF genes are induced by drought and heat stress (Schramm et al., 2008 ; Chen et al., 2010 ), whereas CBF4/DREB1D and DREB3 are induced by ABA treatment under drought stress (Haake et al., 2002 ; Shavrukov et al., 2016 ). Additionally, CBF/DREB1-type and DREB2-type TFs show overlapping functions and mechanized temperature adaptation strategies (Agarwal et al., 2017 ). Most importantly, CBF/DREB TFs regulate all co-regulated genes, which helps to maintain normal photosynthesis, stomatal conductance, Chl content, and ETC under stress conditions, thus improving plant growth and development.
Besides the well-identified role of TFs, some candidate genes also have been recognized as a fast and reliable way to directly link leaf photosynthesis in in crop plants with the level of tolerance to different abiotic stresses (Nawaz et al., 2018 ). Overexpression of the Arabidopsis HARDY ( HRD ) gene in rice enhanced photosynthetic assimilation and reduced the transpiration rate (Karaba et al., 2007 ). ABR17 , a member of pathogenesis-related proteins (PR10), showed increased tolerance lto multiple stresses, and improved the rate of seed germination in Arabidopsis (Srivastava et al., 2004 ). The mitochondrial pentatricopeptide repeat (PPR) proteins play a vital role in post-transcriptional regulation and embryo development (Chintakovid et al., 2017 ). Further studies on the Arabidopsis ppr40 mutant confirmed the importance of PPR proteins in organogenesis, embryo development, and irregular photosynthesis (Pusnik et al., 2007 ; Manna, 2015 ). The regulation of PcINO1 and McIMTI genes in tobacco enhanced salt tolerance and within chloroplasts and cytosol increased the level of inositol, as well as transgenic plants endorse the growth and photosynthesis with minor oxidative damage compared with wild-type plants under salt stress (Patra et al., 2010 ). Mitogen-activated protein kinases (MAPKs) are involved in signal transduction from the chloroplast to the nucleus, chloroplast redox regulated gene expression, metabolic/cellular processes, and response to external stimulus in eukaryotes (Danquah et al., 2014 ; Dietz et al., 2016 ; Raja et al., 2017 ). Constitutive expression of the tobacco NPK1 gene in maize enhanced drought tolerance (Zong et al., 2009 ) and alleviated the rate of photosynthesis under water deficit stress (Zhang et al., 2012 ). Similarly, sugar-mediated regulation of genes enhanced the photosynthetic components in sugar deficient situation (Pego et al., 1999 ), whereas the regulation of genes related to sugar content enhanced the photosynthetic capacity by increasing the level of sugar in leaf, thus activating the photosynthetic components and increasing photosynthesis (Pego et al., 2000 ). Together, these studies suggest that the regulation of specific genes improves the processes of photosynthesis or photosynthesis related machinery.
Biodegradable and Biostimulant Compounds Boost Plant Health and Significantly Improve the Process of Photosynthesis
Excess application of inorganic fertilizers pollutes the environment and probably provokes natural disasters. Therefore, to avoid or escape from this situation, the research interest has shifted to exploring advanced strategies employing biodegradable compounds such as chitosan (CT), beneficial fungi and humic acid (HA) as well as biostimulants (melatonin and bio-waste) to increase plant growth, photosynthesis, nutrition value, soil structure/texture, and stress tolerance (Castro et al., 2012 ; Petrozza et al., 2014 ; Bulgari et al., 2015 ; du Jardin, 2015 ; Saa et al., 2015 ).
CT is produced from chitin, a basic constituent of sea food shells, and shows great application potential, especially under stress conditions (Sharif et al., 2018 ). CTs promote the seed germination rate and facilitate plant nutrient uptake in wheat under salt stress (Zong et al., 2017 ; Li et al., 2019 ). A recent study showed that CT biopolymers efficiently improve plant physiology and gene regulation, and facilitate the activation of plant defense signaling pathways to increase drought stress tolerance (Sharif et al., 2018 ). Similarly, spray application of CTs enhanced plant growth, Chl content, and photosynthetic machinery in B. napus plants under Cd stress (Zong et al., 2017 ). Furthermore, CTs induced ABA activity, which plays an imperative role in the regulation of stomatal aperture to lower the transpiration rate under stress conditions (Turk, 2019 ). Foliar application of CTs (250 mg/L) increased the amount of Chl and total carbohydrates in cowpea (Farouk and Amany, 2012 ); the same affect was observed in maize, soybean and bean using chitin oligosaccharides (Hidangmayum et al., 2019 ; Mukhtar Ahmed et al., 2020 ). Therefore, it can be speculated that might increase in N and K content boost chloroplast cells in shoot, that further enhance Chl synthesis. The aforementioned reports shed light on the beneficial effect of CTs on plant health and photosynthetic machinery under stress conditions.
HA is an organic fertilizer derived from organic waste materials that can enhance plant growth and development. Foliar application of HA markedly increases photosynthesis and Chl content and improves the chloroplast ultrastructure and other morphological features in chrysanthemum ( Chrysanthemum morifolium ) seedlings (Fan et al., 2014 ). In Plantago ovata , application of HA and fulvic acid resulted in noticeable effects on Chl content and photosynthesis under salt stress (Gholami et al., 2013 ). Similarly, in common bean the HA supplements shoot-up plant morphology, biomass, and Chl content under salt stress (Meganid et al., 2015 ).
Melatonin (N-acetyl-5-methoxy tryptamine) is a ubiquitous molecule and most probably synthesized in chloroplasts and mitochondria and then translocated to other plant parts (Arnao and Hernández-Ruiz, 2014 , 2019 ; Kołodziejczyk and Posmyk, 2016 ; Sun et al., 2020 ). In plants, HA performs various biological functions, such as signaling molecule stimulation, photo response regulation, stress tolerance, and circadian rhythm (Reiter et al., 2015 ; Hu et al., 2016 ; Nawaz et al., 2016 ). Melatonin participates in signal transduction and improvement of the photosynthetic machinery in several plant species ( Table 2 ), exogenous application of melatonin induces physiological modifications and resistance against several abiotic stresses (Xu et al., 2016a ; Liang et al., 2018 ). For example, melatonin application enhances high temperature tolerance in cucumber seedlings (Xu et al., 2010 ), cold resistance in tomato (Zhang et al., 2021 ), salt tolerance in rice (Liang et al., 2015 ) and vanadium (V), and salt stress tolerance in watermelon ( Citrullus lanatus ) (Li Z. et al., 2017 ; Nawaz et al., 2018 ). In rice seedlings, melatonin delayed leaf senescence by improving the Chl content of leaves, which reduced the level of ROS either directly or indirectly and promoted antioxidant activity (Liang et al., 2015 ). Similarly, pretreatment of watermelon seeds with melatonin enhanced photosynthate assimilation, Chl content, ROS production, and antioxidant activity in watermelon seedlings (Li Z. et al., 2017 ; Nawaz et al., 2018 ). Melatonin biosynthesis increases the tolerance to salt stress by improving chloroplast structure and the process of photosynthesis (Zheng et al., 2017 ). Moreover, melatonin protects against drought stress by protecting the chloroplast ultrastructure in the spongy mesophyll of grape leaves, thereby improving the efficiency of PSII (Meng et al., 2014 ). Table 2 listed the effect of melatonin on photosynthesis in several crops.
Induced photosynthetic activity and growth attributes by melatonin in various crops under abiotic stresses.
Despite these biostimulant applications, melatonin had prominent role in transgenic crops such as the N-acetylserotonin-O-methyltransferase (ASMT) which is a specific enzyme required for melatonin synthesis. MzASMT1 from apple rootstock ( Malus zumi Mats) was induced by drought stress in apple leaves, while its over-expression in transgenic Arabidopsis , the melatonin levels were 2–4 times higher than those in the wild type which indicates a positive relation of MzASMT1 to melatonin production in drought stress (Zuo et al., 2014 ). The apple SNAT (serotonin N-acetyltransferase, MzSNAT5 ) was highly expressed by drought in transgenic Arabidopsis and elevated the melatonin levels thus enhanced tolerance to drought (Wang L. et al., 2017 ). Likewise, wheat TaCOMT (Caffeic acid 3-O-methyltransferase), gene over-expression enhances drought tolerance and produced higher melatonin, proline, and lower malondialdehyde (MDA) contents, than that in wild type (WT) plants in transgenic Arabidopsis (Yang et al., 2019 ).
Overexpression of N-acetyltransferase1 and human serotonin N-acetyltransferase in transgenic rice confirmed resistance against cold and Cd stress (Kang et al., 2007 ), while overexpression of alfalfa SNAT in Arabidopsis conferred greater resistance to salt stress and increased reestablishment of redox and ion homeostasis compared with the wild type (Zhao et al., 2019 ). Moreover, expression of ovine AANAT and HIOMT genes in switch grass ( Panicum virgatum ) improved plant growth and salt tolerance (Huang et al., 2017 ). However, we still covered the limited application of melatonin in the improvement of photosynthesis under abiotic stresses, but in the light of current contribution of melatonin proved significant clues of photosynthesis and plant growth improvements. Although, we suggest further investigation to identify specific genes regulated by biodegradable and biostimulant treatments that improve the process of photosynthesis.
Conclusion and Future Perspectives
In the current review article, we summarized the research progress made so far in the field of photosynthetic performance under abiotic stresses and several approaches that have been successfully used to increase the productivity and abiotic stress tolerance of plants, although many other challenging ideas are yet to be explored.
Abiotic stresses have a common mode of toxicity in plants which is the production of ROS, causing oxidative damage, and membrane instability. Therefore, genetic manipulation of antioxidants, sufficient knowledge of ROS signaling and its regulatory responses, stress regulatory pathways, and functional characterization of key genes can increase tolerance to a variety of stresses. Although numerous studies have thoroughly discussed the significance of genes involved in abiotic stress, but the specific role in photosynthetic machinery still needs deep investigation. Moreover, approaches toward the improvement of the components of PSII and LHC should be shifted toward the ROS scavenging and antioxidant activity. Additionally, the elucidation of redox signaling pathways related to both ROS and antioxidants could provide much more useful molecular tools for the up-regulation of whole suites of genes with protective functions that could make the photosynthetic system much less susceptible to the light- and ROS-mediated modifications.
Evidence shows that stresses usually depends upon their intensity and duration that either up-regulate or down-regulate the genes involved in the mechanism of photosynthesis in plants. Thus, the obtaining the expression patterns of such genes can help to understand the plant photosynthetic or other metabolic responses to various stresses and further functional validation may enhance the photosynthetic capacity in different crops under stressful conditions. We propose that a series of comprehensive and more logical studies need to be conducted that cover the genomic to proteomic and physiological to biochemical analyses of plants under stress. The transgenic approaches still carry a larger scope for a better crop improvement, where the diverse range of abiotic stresses needed to be tested on the basis of the inducible promoter targeting a particular tissue, stage, and specific environmental stress, this may allow to grow transgenic plants under harsh environment with minimum yield losses as well as help in understating the genetic and environmental interactions.
Moreover, the alteration of photosynthesis by phytohormones under stress is not based on a single event but on a complex process of signaling networks and the expression behavior of phytohormone regulated photosynthetic genes. Here, the putative functions of genes during photosynthesis and the correlation between transcriptomic profiling and phytohormone regulatory networks largely link the valuable data set. It would be helpful to further explain the cellular response to environmental stresses and the transcriptional regulation of photosynthetic metabolism rate.
The redox state is regulated by various environmental factors, and the destabilized redox state reprograms the process of photosynthetic ETC, chloroplast genes and various other metabolic, and signaling responses. Therefore, in future research, the efficiency of light use and photosynthetic electron transport yield, improving carbon assimilation, and limiting its loss during photorespiration are the main points to be addressed. Previously, documented data indicated that photosynthesis efficiency decline by the inhibition of RUBISCO activity, therefore, we suggest that modifications of Rubisco to obtain higher turnover rates where the substrate specificity is not a very promising direction for future research. It would be more useful to focus on three approaches, to improve carbon fixation via genetic engineering, namely improving the catalytic properties of Rubisco, introduction of carbon concentration mechanisms (CCMs) and engineering of photorespiration. This would improve the Rubisco and increase the rate of the RuBP regeneration phase in chloroplast. Although TFs play a major role in abiotic stress responses, the regulation of photosynthetic genes by TFs is not yet fully understood. In addition, the area should be investigated more thoroughly in terms of their significance for photosynthesis improvement, plant productivity, and stress resistance. Overall, data suggests that the enhanced photosynthetic machinery and metabolic signaling with improved stress tolerance in plants necessitate a more comprehensive understanding of the inter-disciplinary strategies based on different areas of research.
Author Contributions
IM conceived the idea, designed the outlines, and wrote the manuscript. AS and MA revised the initial draft. Q-HY and HA revised the final manuscript. FL provided useful ideas and funding. All authors approved the final version of manuscript.
Conflict of Interest
The authors declare that the research was conducted in the absence of any commercial or financial relationships that could be construed as a potential conflict of interest.
Funding. We acknowledge the funding agencies, the study was supported by National Millet Crops Research and Development System (CARS-06-A26), National Natural Science Foundation of China (31371529), National Science and Technology Supporting Plan (2014BAD07B03), and Program for the Key Science and Technology in Shaanxi Province (S2018-YF-TSLNY-0005) and Minor Grain Crops Research.
- Agarie S., Miura A., Sumikura R., Tsukamoto S., Nose A., Arima S., et al. (2002). Overexpression of C4 PEPC caused O 2 -insensitive photosynthesis in transgenic rice plants . Plant Sci . 162 , 257–265. 10.1016/S0168-9452(01)00572-6 [ CrossRef ] [ Google Scholar ]
- Agarwal P. K., Gupta K., Lopato S., Agarwal P. (2017). Dehydration responsive element binding transcription factors and their applications for the engineering of stress tolerance . J. Exp. Bot . 68 , 2135–2148. 10.1093/jxb/erx118 [ PubMed ] [ CrossRef ] [ Google Scholar ]
- Ahmad H., Hayat S., Ali M., Liu H., Chen X., Li J., et al.. (2020). The protective role of 28-homobrassinolide and glomus versiforme in cucumber to withstand saline stress . Plants . 9 :42. 10.3390/plants9010042 [ PMC free article ] [ PubMed ] [ CrossRef ] [ Google Scholar ]
- Ahmad H., Hayat S., Ali M., Liu T., Cheng Z. (2018). The combination of arbuscular mycorrhizal fungi inoculation ( Glomus versiforme ) and 28-homobrassinolide spraying intervals improves growth by enhancing photosynthesis, nutrient absorption, and antioxidant system in cucumber ( Cucumis sativus L.) under salinity . Ecol. Evol . 8 , 5724–5740. 10.1002/ece3.4112 [ PMC free article ] [ PubMed ] [ CrossRef ] [ Google Scholar ]
- Ahmed A., Tajmir-Riahi H. A. (1993). Interaction of toxic metal ions Cd 2+ , Hg 2+ , and Pb 2+ with light-harvesting proteins of chloroplast thylakoid membranes. An FTIR spectroscopic study . J. Inorg. Biochem . 50 , 235–243. 10.1016/0162-0134(93)80050-J [ CrossRef ] [ Google Scholar ]
- Akhtar M., Jaiswal A., Taj G., Jaiswal J. P., Qureshi M. I., Singh N. K. (2012). DREB1/CBF transcription factors: their structure, function and role in abiotic stress tolerance in plants . J. Genet . 91 , 385–395. 10.1007/s12041-012-0201-3 [ PubMed ] [ CrossRef ] [ Google Scholar ]
- Akram M. S., Ashraf M. (2011). Exogenous application of potassium dihydrogen phosphate can alleviate the adverse effects of salt stress on sunflower . J. Plant Nutr . 34 , 1041–1057. 10.1080/01904167.2011.555585 [ CrossRef ] [ Google Scholar ]
- Akram N. A., Ashraf M. (2011). Improvement in growth, chlorophyll pigments and photosynthetic performance in salt-stressed plants of sunflower ( Helianthus animus L.) by foliar application of 5-aminolevulinic acid . Agrochimica Pica 55 , 94–104. [ Google Scholar ]
- Albert K. R., Mikkelsen T. N., Michelsen A., Ro-Poulsen H., van der Linden L. (2011). Interactive effects of drought, elevated CO 2 and warming on photosynthetic capacity and photosystem performance in temperate heath plants . J. Plant Physiol . 168 , 1550–1561. 10.1016/j.jplph.2011.02.011 [ PubMed ] [ CrossRef ] [ Google Scholar ]
- Allen D. J., Ort D. R. (2001). Impacts of chilling temperatures on photosynthesis in warm-climate plants . Trends Plant Sci . 6 , 36–42. 10.1016/S1360-1385(00)01808-2 [ PubMed ] [ CrossRef ] [ Google Scholar ]
- Alonso-Ramírez A., Rodríguez D., Reyes D., Jiménez J. A., Nicolás G., López-Climent M., et al.. (2009). Evidence for a role of gibberellins in salicylic acid-modulated early plant responses to abiotic stress in Arabidopsis seeds . Plant Physiol . 150 , 1335–1344. 10.1104/pp.109.139352 [ PMC free article ] [ PubMed ] [ CrossRef ] [ Google Scholar ]
- Ambavaram M. M. R., Basu S., Krishnan A., Ramegowda V., Batlang U., Rahman L., et al.. (2014). Coordinated regulation of photosynthesis in rice increases yield and tolerance to environmental stress . Nat. Commun . 5 :5302. 10.1038/ncomms6302 [ PMC free article ] [ PubMed ] [ CrossRef ] [ Google Scholar ]
- Anjana J., Allakhverdiev S. I. (2017). High-temperature stress in plants: consequences and strategies for protecting photosynthetic machinery , in Plant Stress Physiology, 2nd Edn , ed Shabala S. (Oxfordshire: CAB International; ) pp 138–154 10.1079/9781780647296.0138 [ CrossRef ] [ Google Scholar ]
- Anjum S. A., Ashraf U., Tanveer M., Khan I., Hussain S., Shahzad B., et al.. (2017). Drought induced changes in growth, osmolyte accumulation and antioxidant metabolism of three maize hybrids . Front. Plant Sci . 8 :69. 10.3389/fpls.2017.00069 [ PMC free article ] [ PubMed ] [ CrossRef ] [ Google Scholar ]
- Anjum S. A., Tanveer M., Ashraf U., Hussain S., Shahzad B., Khan I., et al.. (2016a). Effect of progressive drought stress on growth, leaf gas exchange, and antioxidant production in two maize cultivars . Environ. Sci. Pollut. Res . 23 , 17132–17141. 10.1007/s11356-016-6894-8 [ PubMed ] [ CrossRef ] [ Google Scholar ]
- Anjum S. A., Tanveer M., Hussain S., Bao M., Wang L., Khan I., et al.. (2015). Cadmium toxicity in maize ( Zea mays L.): consequences on antioxidative systems, reactive oxygen species and cadmium accumulation . Environ. Sci. Pollut. Res . 22 , 17022–17030. 10.1007/s11356-015-4882-z [ PubMed ] [ CrossRef ] [ Google Scholar ]
- Anjum S. A., Tanveer M., Hussain S., Shahzad B., Ashraf U., Fahad S., et al.. (2016b). Osmoregulation and antioxidant production in maize under combined cadmium and arsenic stress . Environ. Sci. Pollut. Res . 23 , 11864–11875. 10.1007/s11356-016-6382-1 [ PubMed ] [ CrossRef ] [ Google Scholar ]
- Anjum S. A., Tanveer M., Hussain S., ullah E., Wang L., Khan I., et al. (2016c). Morpho-physiological growth and yield responses of two contrasting maize cultivars to cadmium exposure . Clean Soil Air Water . 44 , 29–36. 10.1002/clen.201400905 [ CrossRef ] [ Google Scholar ]
- Anjum S. A., Xie X., yu Wang L., chang Saleem M. F., Man C., Lei W. (2011). Morphological, physiological and biochemical responses of plants to drought stress . African J. Agric. Res . 6 , 2026–2032. 10.21921/jas.5.3.7 [ CrossRef ] [ Google Scholar ]
- Antoniou C., Chatzimichail G., Xenofontos R., Pavlou J. J., Panagiotou E., Christou A., et al.. (2017). Melatonin systemically ameliorates drought stress-induced damage in Medicago sativa plants by modulating nitro-oxidative homeostasis and proline metabolism . J. Pineal Res . 62 , 1–14. 10.1111/jpi.12401 [ PubMed ] [ CrossRef ] [ Google Scholar ]
- Aragao M., Guedes M., Otoch M., Florindo Guedes M. I., Melo D., Lima M. (2005). Differential responses of ribulose-1,5-bisphosphate carboxylase/oxygenase activities of two Vigna unguiculata cultivars to salt stress . Braz. J. Plant Physiol . 17 , 207–212. 10.1590/S1677-04202005000200003 [ CrossRef ] [ Google Scholar ]
- Arfan M., Athar H. R., Ashraf M. (2007). Does exogenous application of salicylic acid through the rooting medium modulate growth and photosynthetic capacity in two differently adapted spring wheat cultivars under salt stress? J. Plant Physiol . 164 , 685–694. 10.1016/j.jplph.2006.05.010 [ PubMed ] [ CrossRef ] [ Google Scholar ]
- Arnao M. B., Hernández-Ruiz J. (2014). Melatonin: plant growth regulator and/or biostimulator during stress? Trends Plant Sci . 19 , 789–797. 10.1016/j.tplants.2014.07.006 [ PubMed ] [ CrossRef ] [ Google Scholar ]
- Arnao M. B., Hernández-Ruiz J. (2019). Melatonin: a new plant hormone and/or a plant master regulator? Trends Plant Sci . 24 , 38–48. 10.1016/j.tplants.2018.10.010 [ PubMed ] [ CrossRef ] [ Google Scholar ]
- Ashraf M. (1994). Breeding for salinity tolerance in plants . CRC. Crit. Rev. Plant Sci . 13 , 17–42. 10.1080/07352689409701906 [ CrossRef ] [ Google Scholar ]
- Ashraf M., Ali Q. (2008). Relative membrane permeability and activities of some antioxidant enzymes as the key determinants of salt tolerance in canola ( Brassica napus L.) . Environ. Exp. Bot . 63 , 266–273. 10.1016/j.envexpbot.2007.11.008 [ CrossRef ] [ Google Scholar ]
- Ashraf M., Harris P. J. C. (2013). Photosynthesis under stressful environments: an overview . Photosynthetica 51 , 163–190. 10.1007/s11099-013-0021-6 [ CrossRef ] [ Google Scholar ]
- Ashraf M., Mehmood S. (1990). Response of four brassica species to drought stress . Environ. Exp. Bot . 30 , 93–100. 10.1016/0098-8472(90)90013-T [ CrossRef ] [ Google Scholar ]
- Ashraf M., Sultana R. (2000). Combination effect of NaCl salinity and nitrogen form on mineral composition of sunflower plants . Biol. Plant . 43 , 615–619. 10.1023/A:1002860202032 [ CrossRef ] [ Google Scholar ]
- Attaran E., Major I. T., Cruz J. A., Rosa B. A., Koo A. J. K., Chen J., et al.. (2014). Temporal dynamics of growth and photosynthesis suppression in response to jasmonate signaling . Plant Physiol . 165 , 1302–1314. 10.1104/pp.114.239004 [ PMC free article ] [ PubMed ] [ CrossRef ] [ Google Scholar ]
- Bagur M. G., Morales S., López-Chicano M. (2009). Evaluation of the environmental contamination at an abandoned mining site using multivariate statistical techniques-the rodalquilar (Southern Spain) mining district . Talanta . 80 , 377–384. 10.1016/j.talanta.2009.06.075 [ PubMed ] [ CrossRef ] [ Google Scholar ]
- Bai M. Y., Shang J. X., Oh E., Fan M., Bai Y., Zentella R., et al.. (2012). Brassinosteroid, gibberellin and phytochrome impinge on a common transcription module in Arabidopsis . Nat. Cell Biol . 14 , 810–817. 10.1038/ncb2546 [ PMC free article ] [ PubMed ] [ CrossRef ] [ Google Scholar ]
- Balouchi H. R. (2010). Screening wheat parents of mapping population for heat and drought tolerance, detection of wheat genetic variation . World Acad. Sci. Eng. Technol . 4 , 63–73. 10.5281/zenodo.1058455 [ CrossRef ] [ Google Scholar ]
- Bao G., Zhuo C., Qian C., Xiao T., Guo Z., Lu S. (2016). Co-expression of NCED and ALO improves vitamin C level and tolerance to drought and chilling in transgenic tobacco and stylo plants . Plant Biotechnol. J . 14 , 206–214. 10.1111/pbi.12374 [ PubMed ] [ CrossRef ] [ Google Scholar ]
- Barickman T. C., Kopsell D. A., Sams C. E. (2014). Abscisic acid increases carotenoid and chlorophyll concentrations in leaves and fruit of two tomato genotypes . J. Am. Soc. Hortic. Sci . 139 , 261–266. 10.21273/jashs.139.3.261 [ CrossRef ] [ Google Scholar ]
- Barros T., Royant A., Standfuss J., Dreuw A., Kühlbrandt W. (2009). Crystal structure of plant light-harvesting complex shows the active, energy-transmitting state . EMBO J . 28 , 298–306. 10.1038/emboj.2008.276 [ PMC free article ] [ PubMed ] [ CrossRef ] [ Google Scholar ]
- Barth C., Krause G. H., Winter K. (2001). Responses of photosystem I compared with photosystem II to high-light stress in tropical shade and sun leaves . Plant Cell Environ . 24 , 163–176. 10.1046/j.1365-3040.2001.00673.x [ CrossRef ] [ Google Scholar ]
- Bartoli C. G., Casalongué C. A., Simontacchi M., Marquez-Garcia B., Foyer C. H. (2013). Interactions between hormone and redox signalling pathways in the control of growth and cross tolerance to stress . Environ. Exp. Bot . 94 , 73–88. 10.1016/j.envexpbot.2012.05.003 [ CrossRef ] [ Google Scholar ]
- Batra N. G., Sharma V., Kumari N. (2014). Drought-induced changes in chlorophyll fluorescence, photosynthetic pigments, and thylakoid membrane proteins of Vigna radiata . J. Plant Interact . 9 , 712–721. 10.1080/17429145.2014.905801 [ CrossRef ] [ Google Scholar ]
- Berenschot A. S., Quecini V. (2014). A reverse genetics approach identifies novel mutants in light responses and anthocyanin metabolism in petunia . Physiol. Mol. Biol. Plants . 20 , 1–13. 10.1007/s12298-013-0212-4 [ PMC free article ] [ PubMed ] [ CrossRef ] [ Google Scholar ]
- Bertioli D. J., Cannon S. B., Froenicke L., Huang G., Farmer A. D., Cannon E. K. S., et al.. (2016). The genome sequences of arachis duranensis and arachis ipaensis, the diploid ancestors of cultivated peanut . Nat. Genet . 48 , 438–446. 10.1038/ng.3517 [ PubMed ] [ CrossRef ] [ Google Scholar ]
- Biswal B., Joshi P. N., Raval M. K., Biswal U. C. (2011). Photosynthesis, a global sensor of environmental stress in green plants: stress signalling and adaptation . Curr. Sci . 101 , 47–56. [ Google Scholar ]
- Boisvert S., Joly D., Leclerc S., Govindachary S., Harnois J., Carpentier R. (2007). Inhibition of the oxygen-evolving complex of photosystem II and depletion of extrinsic polypeptides by nickel . BioMetals . 20 , 879–889. 10.1007/s10534-007-9081-z [ PubMed ] [ CrossRef ] [ Google Scholar ]
- Budak H., Akpinar B. A., Unver T., Turktas M. (2013). Proteome changes in wild and modern wheat leaves upon drought stress by two-dimensional electrophoresis and nanoLC-ESI-MS/MS . Plant Mol. Biol . 83 , 89–103. 10.1007/s11103-013-0024-5 [ PubMed ] [ CrossRef ] [ Google Scholar ]
- Bulgari R., Cocetta G., Trivellini A., Vernieri P., Ferrante A. (2015). Biostimulants and crop responses: a review . Biol. Agric. Hortic . 31 , 1–17. 10.1080/01448765.2014.964649 [ CrossRef ] [ Google Scholar ]
- Burkhead J. L., Gogolin Reynolds K. A., Abdel-Ghany S. E., Cohu C. M., Pilon M. (2009). Copper homeostasis . New Phytol . 182 , 799–816. 10.1111/j.1469-8137.2009.02846.x [ PubMed ] [ CrossRef ] [ Google Scholar ]
- Castro J., Vera J., González A., Moenne A. (2012). Oligo-carrageenans stimulate growth by enhancing photosynthesis, basal metabolism, and cell cycle in tobacco plants (var. Burley) . J. Plant Growth Regul . 31 , 173–185. 10.1007/s00344-011-9229-5 [ CrossRef ] [ Google Scholar ]
- Centritto M., Brilli F., Fodale R., Loreto F. (2011). Different sensitivity of isoprene emission, respiration and photosynthesis to high growth temperature coupled with drought stress in black poplar ( Populus nigra ) saplings . Tree Physiol . 31 , 275–286. 10.1093/treephys/tpq112 [ PubMed ] [ CrossRef ] [ Google Scholar ]
- Chaves M. M., Flexas J., Pinheiro C. (2009). Photosynthesis under drought and salt stress: regulation mechanisms from whole plant to cell . Ann. Bot . 103 , 551–560. 10.1093/aob/mcn125 [ PMC free article ] [ PubMed ] [ CrossRef ] [ Google Scholar ]
- Chen H., Hwang J. E., Lim C. J., Kim D. Y., Lee S. Y., Lim C. O. (2010). Arabidopsis DREB2C functions as a transcriptional activator of HsfA3 during the heat stress response . Biochem. Biophys. Res. Commun . 401 , 238–244. 10.1016/j.bbrc.2010.09.038 [ PubMed ] [ CrossRef ] [ Google Scholar ]
- Chen L., Jia H., Tian Q., Du L., Gao Y., Miao X., et al.. (2012). Protecting effect of phosphorylation on oxidative damage of D1 protein by down-regulating the production of superoxide anion in photosystem II membranes under high light . Photosynth. Res . 112 , 141–148. 10.1007/s11120-012-9750-9 [ PubMed ] [ CrossRef ] [ Google Scholar ]
- Chen Y., Zhou B., Li J., Tang H., Tang J., Yang Z. (2018). Formation and change of chloroplast-located plant metabolites in response to light conditions . Int. J. Mol. Sci . 19 :654. 10.3390/ijms19030654 [ PMC free article ] [ PubMed ] [ CrossRef ] [ Google Scholar ]
- Cheng Z., Dong K., Ge P., Bian Y., Dong L., Deng X., et al.. (2015). Identification of leaf proteins differentially accumulated between wheat cultivars distinct in their levels of drought tolerance . PLoS ONE 10 :e0125302. 10.1371/journal.pone.0125302 [ PMC free article ] [ PubMed ] [ CrossRef ] [ Google Scholar ]
- Cherif J., Mediouni C., Ammar W. B., Jemal F. (2011). Interactions of zinc and cadmium toxicity in their effects on growth and in antioxidative systems in tomato plants ( Solarium lycopersicum ) . J. Environ. Sci . 23 , 837–844. 10.1016/S1001-0742(10)60415-9 [ PubMed ] [ CrossRef ] [ Google Scholar ]
- Chintakovid N., Maipoka M., Phaonakrop N., Mickelbart M. V., Roytrakul S., Chadchawan S. (2017). Proteomic analysis of drought-responsive proteins in rice reveals photosynthesis-related adaptations to drought stress . Acta Physiol. Plant . 39 :240 10.1007/s11738-017-2532-4 [ CrossRef ] [ Google Scholar ]
- Chinthapalli B., Murmu J., Raghavendra A. S. (2003). Dramatic difference in the responses of phosphoenolpyruvate carboxylase to temperature in leaves of C3 and C4 plants . J. Exp. Bot . 54 , 707–714. 10.1093/jxb/erg078 [ PubMed ] [ CrossRef ] [ Google Scholar ]
- Choudhary S. P., Yu J. Q., Yamaguchi-Shinozaki K., Shinozaki K., Tran L. S. P. (2012). Benefits of brassinosteroid crosstalk . Trends Plant Sci . 17 , 594–605. 10.1016/j.tplants.2012.05.012 [ PubMed ] [ CrossRef ] [ Google Scholar ]
- Cortleven A., Nitschke S., Klaumünzer M., AbdElgawad H., Asard H., Grimm B., et al.. (2014). A novel protective function for cytokinin in the light stress response is mediated by the arabidopsis histidine kinase2 and arabidopsis histidine kinase3 receptors . Plant Physiol . 164 , 1470–1483. 10.1104/pp.113.224667 [ PMC free article ] [ PubMed ] [ CrossRef ] [ Google Scholar ]
- Cowie B. W., Byrne M. J., Witkowski E. T. F., Venter N. (2016). Exacerbation of photosynthetic damage through increased heat-light stress resulting from Gargaphia decoris sap-feeding . Biol. Control . 94 , 82–89. 10.1016/j.biocontrol.2015.12.008 [ CrossRef ] [ Google Scholar ]
- Crafts-Brandner S. J., Salvucci M. E. (2002). Sensitivity of photosynthesis in a C4 plant, maize, to heat stress . Plant Physiol . 129 :1773. 10.1104/pp.002170 [ PMC free article ] [ PubMed ] [ CrossRef ] [ Google Scholar ]
- Crafts-Brandner S. J., Salvucci M. E. (2004). Analyzing the impact of high temperature and CO2 on net photosynthesis: biochemical mechanisms, models and genomics . Field Crops Res . 90 , 75–85. 10.1016/j.fcr.2004.07.006 [ CrossRef ] [ Google Scholar ]
- Crafts-Brandner S. J., Van De Loo F. J., Salvucci M. E. (1997). The two forms of ribulose-1,5-bisphosphate carboxylase/oxygenase activase differ in sensitivity to elevated temperature . Plant Physiol . 114 , 439–444. 10.1104/pp.114.2.439 [ PMC free article ] [ PubMed ] [ CrossRef ] [ Google Scholar ]
- Cui G., Zhao X., Liu S., Sun F., Zhang C., Xi Y. (2017). Beneficial effects of melatonin in overcoming drought stress in wheat seedlings . Plant Physiol. Biochem . 118 , 138–149. 10.1016/j.plaphy.2017.06.014 [ PubMed ] [ CrossRef ] [ Google Scholar ]
- Czarnocka W., Karpiński S. (2018). Friend or foe? Reactive oxygen species production, scavenging and signaling in plant response to environmental stresses . Free Radic. Biol. Med . 122 , 4–20. 10.1016/j.freeradbiomed.2018.01.011 [ PubMed ] [ CrossRef ] [ Google Scholar ]
- Damayanthi M., Mohotti A., Nissanka S. (2011). Comparison of tolerant ability of mature field grown tea ( Camellia sinensis L.) cultivars exposed to a drought stress in passara area . Trop. Agric. Res . 22 , 66–75. 10.4038/tar.v22i1.2671 [ CrossRef ] [ Google Scholar ]
- Danquah A., de Zelicourt A., Colcombet J., Hirt H. (2014). The role of ABA and MAPK signaling pathways in plant abiotic stress responses . Biotechnol. Adv . 321 , 40–52. 10.1016/j.biotechadv.2013.09.006 [ PubMed ] [ CrossRef ] [ Google Scholar ]
- De Silva N. D. G., Cholewa E., Ryser P. (2012). Effects of combined drought and heavy metal stresses on xylem structure and hydraulic conductivity in red maple ( Acer rubrum L.) . J. Exp. Bot . 63 , 5957–5966. 10.1093/jxb/ers241 [ PubMed ] [ CrossRef ] [ Google Scholar ]
- Demmig-Adams B., Adams W. W. (1992). Photoprotection and other responses of plants to high light stress . Annu. Rev. Plant Physiol. Plant Mol. Biol . 43 , 599–626. 10.1146/annurev.pp.43.060192.003123 [ CrossRef ] [ Google Scholar ]
- Demmig-Adams B., Stewart J. J., Baker C. R., Adams W. W. (2018). Optimization of photosynthetic productivity in contrasting environments by regulons controlling plant form and function . Int. J. Mol. Sci . 19 :872. 10.3390/ijms19030872 [ PMC free article ] [ PubMed ] [ CrossRef ] [ Google Scholar ]
- Dias M. C., Brüggemann W. (2010). Limitations of photosynthesis in Phaseolus vulgaris under drought stress: gas exchange, chlorophyll fluorescence and Calvin cycle enzymes . Photosynthetica 48 , 96–102. 10.1007/s11099-010-0013-8 [ CrossRef ] [ Google Scholar ]
- Dietz K. J., Turkan I., Krieger-Liszkay A. (2016). Redox- and reactive oxygen species-dependent signaling into and out of the photosynthesizing chloroplast . Plant Physiol . 171 , 1541–1550. 10.1104/pp.16.00375 [ PMC free article ] [ PubMed ] [ CrossRef ] [ Google Scholar ]
- Din J., Khan S. U., Ali I., Gurmani A. R. (2011). Physiological and agronomic response of canola varieties to drought stress . J. Anim. Plant Sci . 21 , 79–83. [ Google Scholar ]
- Dobrikova A. G., Vladkova R. S., Rashkov G. D., Todinova S. J., Krumova S. B., Apostolova E. L. (2014). Effects of exogenous 24-epibrassinolide on the photosynthetic membranes under non-stress conditions . Plant Physiol. Biochem . 80 , 75–82. 10.1016/j.plaphy.2014.03.022 [ PubMed ] [ CrossRef ] [ Google Scholar ]
- Donohue R. J., Roderick M. L., McVicar T. R., Farquhar G. D. (2013). Impact of CO2 fertilization on maximum foliage cover across the globe's warm, arid environments . Geophys. Res. Lett . 40 , 3031–3035. 10.1002/grl.50563 [ CrossRef ] [ Google Scholar ]
- Drazkiewicz M. (1994). Chlorophyllase: occurrence, functions, mechanism of action, effects of external and internal factors . Photosynthetica 30 , 321–330. [ Google Scholar ]
- Drzewiecka K., Borowiak K., Bandurska H., Golinski P. (2012). Salicylic acid - A potential biomarker of tobacco Bel-W3 cell death developed as a response to ground level ozone under ambient conditions . Acta Biol. Hung . 63 , 231–249. 10.1556/ABiol.63.2012.2.6 [ PubMed ] [ CrossRef ] [ Google Scholar ]
- du Jardin P. (2015). Plant biostimulants: definition, concept, main categories and regulation . Sci. Hortic . 196 , 3–14. 10.1016/j.scienta.2015.09.021 [ CrossRef ] [ Google Scholar ]
- Du Y. C., Nose A., Wasano K., Uchida Y. (1998). Responses to water stress of enzyme activities and metabolite levels in relation to sucrose and starch synthesis, the Calvin cycle and the C4 pathway in sugarcane ( Saccharum sp.) leaves . Aust. J. Plant Physiol . 25 , 253–260. 10.1071/PP97015 [ CrossRef ] [ Google Scholar ]
- Dutta S., Mohanty S., Tripathy B. C. (2009). Role of temperature stress on chloroplast biogenesis and protein import in pea1[OA] . Plant Physiol . 150 , 1050–1061. 10.1104/pp.109.137265 [ PMC free article ] [ PubMed ] [ CrossRef ] [ Google Scholar ]
- Eckardt N. A. (2009). A new chlorophyll degradation pathway . Plant Cell . 21 :700. 10.1105/tpc.109.210313 [ PMC free article ] [ PubMed ] [ CrossRef ] [ Google Scholar ]
- Efeoglu B., Terzioglu S. (2009). Photosynthetic responses of two wheat varieties to high temperature . EurAsian J. Biosci . 3 , 97–106. 10.5053/ejobios.2009.3.0.13 [ CrossRef ] [ Google Scholar ]
- Elsheery N. I., Cao K. F. (2008). Gas exchange, chlorophyll fluorescence, and osmotic adjustment in two mango cultivars under drought stress . Acta Physiol. Plant . 30 , 769–777. 10.1007/s11738-008-0179-x [ CrossRef ] [ Google Scholar ]
- El-Tayeb M. A. (2005). Response of barley grains to the interactive effect of salinity and salicylic acid . Plant Growth Regul . 45 , 215–224. 10.1007/s10725-005-4928-1 [ CrossRef ] [ Google Scholar ]
- Erickson E., Wakao S., Niyogi K. K. (2015). Light stress and photoprotection in Chlamydomonas reinhardtii . Plant J . 82 , 449–465. 10.1111/tpj.12825 [ PubMed ] [ CrossRef ] [ Google Scholar ]
- Evans J. R. (2013). Improving photosynthesis . Plant Physiol . 162 , 1780–1793. 10.1104/pp.113.219006 [ PMC free article ] [ PubMed ] [ CrossRef ] [ Google Scholar ]
- Falk J., Munné-Bosch S. (2010). Tocochromanol functions in plants: antioxidation and beyond . J. Exp. Bot . 61 , 1549–1566. 10.1093/jxb/erq030 [ PubMed ] [ CrossRef ] [ Google Scholar ]
- Fan H. M., Wang X. W., Sun X., Li Y. Y., Sun X. Z., Zheng C. A., et al. (2014). Effects of humic acid derived from sediments on growth, photosynthesis and chloroplast ultrastructure in chrysanthemum . Sci. Horticulturae 177 , 118–123. 10.1016/j.scienta.2014.05.010 [ CrossRef ] [ Google Scholar ]
- Farouk S., Amany A. (2012). Improving growth and yield of cowpea by foliar application of chitosan under water stress . Egypt. J. Biol . 14 , 14–16. 10.4314/ejb.v14i1.2 [ CrossRef ] [ Google Scholar ]
- Faseela P., Puthur J. T. (2017). Chlorophyll a fluorescence changes in response to short and long term high light stress in rice seedlings . Indian J. Plant Physiol . 22 , 30–33. 10.1007/s40502-016-0244-1 [ CrossRef ] [ Google Scholar ]
- Faseela P., Puthur J. T. (2018). The imprints of the high light and UV-B stresses in Oryza sativa L. ‘Kanchana’ seedlings are differentially modulated . J. Photochem. Photobiol. B Biol . 178 , 551–559. 10.1016/j.jphotobiol.2017.12.009 [ PubMed ] [ CrossRef ] [ Google Scholar ]
- Filek M., Kościelniak J., Łabanowska M., Bednarska E., Bidzińska E. (2010). Selenium-induced protection of photosynthesis activity in rape ( Brassica napus ) seedlings subjected to cadmium stress. Fluorescence and EPR measurements . Photosynth. Res . 105 , 27–37. 10.1007/s11120-010-9551-y [ PubMed ] [ CrossRef ] [ Google Scholar ]
- Gajewska E., Skłodowska M., Słaba M., Mazur J. (2006). Effect of nickel on antioxidative enzyme activities, proline and chlorophyll contents in wheat shoots . Biol. Plant . 50 , 653–659. 10.1007/s10535-006-0102-5 [ CrossRef ] [ Google Scholar ]
- Gharechahi J., Hajirezaei M. R., Salekdeh G. H. (2015). Comparative proteomic analysis of tobacco expressing cyanobacterial flavodoxin and its wild type under drought stress . J. Plant Physiol . 175 :48–58. 10.1016/j.jplph.2014.11.001 [ PubMed ] [ CrossRef ] [ Google Scholar ]
- Gholami H., Samavat S., Ardebili Z. O. (2013). The alleviating effects of humic substances on photosynthesis and yield of Plantago ovate in salinity conditions . Int. Res. J. Appl. Basic Sci . 4 , 1683–1686. [ Google Scholar ]
- Ghori N. H., Ghori T., Hayat M. Q., Imadi S. R., Gul A., Altay V., et al. (2019). Heavy metal stress and responses in plants . Int. J. Environ. Sci. Technol . 16 , 1807–1828. 10.1007/s13762-019-02215-8 [ CrossRef ] [ Google Scholar ]
- Ghosh S., Bagchi S., Lahiri Majumder A. (2001). Chloroplast fructose-1,6-bisphosphatase from Oryza differs in salt tolerance property from the Porteresia enzyme and is protected by osmolytes . Plant Sci . 160 , 1171–1181. 10.1016/S0168-9452(01)00361-2 [ PubMed ] [ CrossRef ] [ Google Scholar ]
- Gill S. S., Khan N. A., Tuteja N. (2011). Differential cadmium stress tolerance in five indian mustard ( Brassica juncea L.) cultivars: an evaluation of the role of antioxidant machinery . Plant Signal. Behav . 6 , 293–300. 10.4161/psb.6.2.15049 [ PMC free article ] [ PubMed ] [ CrossRef ] [ Google Scholar ]
- Gimenez C., Mitchell V. J., Lawlor D. W. (1992). Regulation of photosynthetic rate of two sunflower hybrids under water stress . Plant Physiol . 98 , 516–524. 10.1104/pp.98.2.516 [ PMC free article ] [ PubMed ] [ CrossRef ] [ Google Scholar ]
- Gomathi R., Rakkiyapan P. (2011). Comparative lipid peroxidation, leaf membrane thermostability, and antioxidant system in four sugarcane genotypes differing in salt tolerance . Int. J. Plant Physiol. Biochem . 3 , 67–74. [ Google Scholar ]
- González-Pérez S., Gutiérrez J., García-García F., Osuna D., Dopazo J., Lorenzo Ó., et al.. (2011). Early transcriptional defense responses in arabidopsis cell suspension culture under high-light conditions . Plant Physiol . 156 , 1439–1456. 10.1104/pp.111.177766 [ PMC free article ] [ PubMed ] [ CrossRef ] [ Google Scholar ]
- Guo H., Chen H., Hong C., Jiang D., Zheng B. (2017a). Exogenous malic acid alleviates cadmium toxicity in Miscanthus sacchariflorus through enhancing photosynthetic capacity and restraining ROS accumulation . Ecotoxicol. Environ. Saf . 141 , 119–128. 10.1016/j.ecoenv.2017.03.018 [ PubMed ] [ CrossRef ] [ Google Scholar ]
- Guo H., Feng X., Hong C., Chen H., Zeng F., Zheng B., et al.. (2017b). Malate secretion from the root system is an important reason for higher resistance of Miscanthus sacchariflorus to cadmium . Physiol. Plant . 159 , 340–353. 10.1111/ppl.12526 [ PubMed ] [ CrossRef ] [ Google Scholar ]
- Guo H., Hong C., Xiao M., Chen X., Chen H., Zheng B., et al.. (2016). Real-time kinetics of cadmium transport and transcriptomic analysis in low cadmium accumulator Miscanthus sacchariflorus . Planta 244 , 1289–1302. 10.1007/s00425-016-2578-3 [ PubMed ] [ CrossRef ] [ Google Scholar ]
- Gururani M. A., Venkatesh J., Tran L. S. P. (2015). Regulation of photosynthesis during abiotic stress-induced photoinhibition . Mol. Plant 8 , 1304–1320. 10.1016/j.molp.2015.05.005 [ PubMed ] [ CrossRef ] [ Google Scholar ]
- Ha C., Van Leyva-Gonzalez M. A., Osakabe Y., Tran U. T., Nishiyama R., Watanabe Y., et al.. (2014). Positive regulatory role of strigolactone in plant responses to drought and salt stress . Proc. Natl. Acad. Sci. U.S.A . 111 , 851–856. 10.1073/pnas.1322135111 [ PMC free article ] [ PubMed ] [ CrossRef ] [ Google Scholar ]
- Haake V., Cook D., Riechmann J. L., Pineda O., Thomashow M. F., Zhang J. Z. (2002). Transcription factor CBF4 is a regulator of drought adaptation in Arabidopsis . Plant Physiol . 130 , 639–648. 10.1104/pp.006478 [ PMC free article ] [ PubMed ] [ CrossRef ] [ Google Scholar ]
- Hajiboland R., Cheraghvareh L., Poschenrieder C. (2017). Improvement of drought tolerance in tobacco ( Nicotiana rustica L.) plants by silicon . J. Plant Nutr . 40 , 1661–1676. 10.1080/01904167.2017.1310887 [ CrossRef ] [ Google Scholar ]
- Hamada A. M., Al-Hakimi A. M. A. (2001). Salicylic acid versus salinity-drought-induced stress on wheat seedlings . Rostl. Vyroba 47 , 444–450. [ Google Scholar ]
- Handa N., Kohli S. K., Sharma A., Thukral A. K., Bhardwaj R., Alyemeni M. N., et al. (2018). Selenium ameliorates chromium toxicity through modifications in pigment system, antioxidative capacity, osmotic system, and metal chelators in Brassica juncea seedlings . South African J. Bot . 119 , 1–10. 10.1016/j.sajb.2018.08.003 [ CrossRef ] [ Google Scholar ]
- Hassinen V. H., Tervahauta A. I., Schat H., Kärenlampi S. O. (2011). Plant metallothioneins - metal chelators with ROS scavenging activity? Plant Biol . 13 , 225–232. 10.1111/j.1438-8677.2010.00398.x [ PubMed ] [ CrossRef ] [ Google Scholar ]
- Häusler R. E., Hirsch H. J., Kreuzaler F., Peterhänsel C. (2002). Overexpression of C4-cycle enzymes in transgenic C3 plants: a biotechnological approach to improve C3-photosynthesis . J. Exp. Bot . 53 , 591–607. 10.1093/jexbot/53.369.591 [ PubMed ] [ CrossRef ] [ Google Scholar ]
- Hernández I. G., Gomez F. J. V., Cerutti S., Arana M. V., Silva M. F. (2015). Melatonin in Arabidopsis thaliana acts as plant growth regulator at low concentrations and preserves seed viability at high concentrations . Plant Physiol. Biochem . 94 , 191–196. 10.1016/j.plaphy.2015.06.011 [ PubMed ] [ CrossRef ] [ Google Scholar ]
- Hidangmayum A., Dwivedi P., Katiyar D., Hemantaranjan A. (2019). Application of chitosan on plant responses with special reference to abiotic stress . Physiol. Mol. Biol. Plants 25 , 313–326. 10.1007/s12298-018-0633-1 [ PMC free article ] [ PubMed ] [ CrossRef ] [ Google Scholar ]
- Hildebrandt T., Knuesting J., Berndt C., Morgan B., Scheibe R. (2015). Cytosolic thiol switches regulating basic cellular functions: GAPDH as an information hub? Biol. Chem . 396 , 523–537. 10.1515/hsz-2014-0295 [ PubMed ] [ CrossRef ] [ Google Scholar ]
- Hirel B., Le Gouis J., Ney B., Gallais A. (2007). The challenge of improving nitrogen use efficiency in crop plants: towards a more central role for genetic variability and quantitative genetics within integrated approaches . J. Exp. Bot . 58 , 2369–2387. 10.1093/jxb/erm097 [ PubMed ] [ CrossRef ] [ Google Scholar ]
- Hou W., Sun A. H., Chen H. L., Yang F. S., Pan J. L., Guan M. Y. (2016). Effects of chilling and high temperatures on photosynthesis and chlorophyll fluorescence in leaves of watermelon seedlings . Biol. Plant 60 , 148–154. 10.1007/s10535-015-0575-1 [ CrossRef ] [ Google Scholar ]
- Hu W., Kong H., Guo Y., Zhang Y., Ding Z., Tie W., et al.. (2016). Comparative physiological and transcriptomic analyses reveal the actions of melatonin in the delay of postharvest physiological deterioration of cassava . Front. Plant Sci . 7 :736. 10.3389/fpls.2016.00736 [ PMC free article ] [ PubMed ] [ CrossRef ] [ Google Scholar ]
- Hu W. H., Yan X. H., Xiao Y. A., Zeng J. J., Qi H. J., Ogweno J. O. (2013). 24-Epibrassinosteroid alleviate drought-induced inhibition of photosynthesis in Capsicum annuum . Sci. Hortic . 150 , 232–237. 10.1016/j.scienta.2012.11.012 [ CrossRef ] [ Google Scholar ]
- Huang W., Hu H., Zhang S. B. (2015a). Photorespiration plays an important role in the regulation of photosynthetic electron flow under fluctuating light in tobacco plants grown under full sunlight . Front. Plant Sci . 6 :621. 10.3389/fpls.2015.00621 [ PMC free article ] [ PubMed ] [ CrossRef ] [ Google Scholar ]
- Huang W., Ma H. Y., Huang Y., Li Y., Wang G. L., Jiang Q., et al.. (2017). Comparative proteomic analysis provides novel insights into chlorophyll biosynthesis in celery under temperature stress . Physiol. Plant . 161 , 468–485. 10.1111/ppl.12609 [ PubMed ] [ CrossRef ] [ Google Scholar ]
- Huang W., Zhang S. B., Hu H. (2015b). Insusceptibility of oxygen-evolving complex to high light in betula platyphylla . J. Plant Res . 6 , 621. 10.1007/s10265-014-0684-5 [ PubMed ] [ CrossRef ] [ Google Scholar ]
- Huerta L., Forment J., Gadea J., Fagoaga C., Peña L., Pérez-Amador M. A., et al.. (2008). Gene expression analysis in citrus reveals the role of gibberellins on photosynthesis and stress . Plant Cell Environ . 31 , 1620–1633. 10.1111/j.1365-3040.2008.01870.x [ PubMed ] [ CrossRef ] [ Google Scholar ]
- Hui D., Yu C. L., Deng Q., Kudjo Dzantor E., Zhou S., Dennis S., et al.. (2018). Effects of precipitation changes on switchgrass photosynthesis, growth, and biomass: a mesocosm experiment . PLoS ONE 13 :e0192555 10.1371/journal.pone.0192555 [ PMC free article ] [ PubMed ] [ CrossRef ] [ Google Scholar ]
- Ivanov A., Velitchkova M. (2014). Chapter 27: Mechanisms of stimulation of photosystem I activity in chloroplast membranes under heat stress. Correlation Between P700 Photooxidation and Thermostability of Thylakoid Membrane Organization , in Contemporary Problems of Photosynthesis, Vol. 2 , eds Allakhverdiev S. I., Rubin A. B., Shuvalov V. A. (Moscow–Izhevsk: Izhevsk Institute of Computer Science; ), 377–395. [ Google Scholar ]
- Ivanov A. G., Kitcheva M. I., Christov A. M., Popova L. P. (1992). Effects of abscisic acid treatment on the thermostability of the photosynthetic apparatus in barley chloroplasts . Plant Physiol . 98 , 1228–1232. 10.1104/pp.98.4.1228 [ PMC free article ] [ PubMed ] [ CrossRef ] [ Google Scholar ]
- Jain M., Tiwary S., Gadre R. (2010). Sorbitol-induced changes in various growth and biochemical parameters in maize . Plant Soil Environ . 56 , 263–267. 10.17221/233/2009-pse [ CrossRef ] [ Google Scholar ]
- Jaleel C. A., Manivannan P., Wahid A., Farooq M., Al-Juburi H. J., Somasundaram R., et al. (2009). Drought stress in plants: a review on morphological characteristics and pigments composition . Int. J. Agric. Biol . 11 , 100–105. [ Google Scholar ]
- Janik E., Maksymiec W., Mazur R., Garstka M., Gruszecki W. I. (2010). Structural and functional modifications of the major light-harvesting complex II in cadmium-or copper-treated Secale cereale . Plant Cell Physiol . 51 , 1330–1340. 10.1093/pcp/pcq093 [ PubMed ] [ CrossRef ] [ Google Scholar ]
- Janka E., Körner O., Rosenqvist E., Ottosen C. O. (2013). High temperature stress monitoring and detection using chlorophyll a fluorescence and infrared thermography in chrysanthemum ( Dendranthema grandiflora ) . Plant Physiol. Biochem . 67 , 87–94. 10.1016/j.plaphy.2013.02.025 [ PubMed ] [ CrossRef ] [ Google Scholar ]
- Jeanneau M., Gerentes D., Foueillassar X., Zivy M., Vidal J., Toppan A., et al.. (2002). Improvement of drought tolerance in maize: towards the functional validation of the Zm-Asr1 gene and increase of water use efficiency by over-expressing C4-PEPC . Biochimie 84 , 1127–1135. 10.1016/S0300-9084(02)00024-X [ PubMed ] [ CrossRef ] [ Google Scholar ]
- Jordan D. B., Ogren W. L. (1984). The CO2/O2 specificity of ribulose 1,5-bisphosphate carboxylase/oxygenase . Planta 161 , 308–313. 10.1007/BF00398720 [ PubMed ] [ CrossRef ] [ Google Scholar ]
- Juan M., Rivero R. M., Romero L., Ruiz J. M. (2005). Evaluation of some nutritional and biochemical indicators in selecting salt-resistant tomato cultivars . Environ. Exp. Bot . 54 , 193–201. 10.1016/j.envexpbot.2004.07.004 [ CrossRef ] [ Google Scholar ]
- Kaewsuksaeng S. (2011). Chlorophyll degradation in horticultural crops . Walailak J. Sci. Tech . 8 , 9–19. 10.2004/WJST.V8I1.7 [ CrossRef ] [ Google Scholar ]
- Kalaji H. M., Jajoo A., Oukarroum A., Brestic M., Zivcak M., Samborska I. A., et al. (2016). Chlorophyll a fluorescence as a tool to monitor physiological status of plants under abiotic stress conditions . Acta Physiol. Plant . 38 :102 10.1007/s11738-016-2113-y [ CrossRef ] [ Google Scholar ]
- Kang S., Kang K., Lee K., Back K. (2007). Characterization of tryptamine 5-hydroxylase and serotonin synthesis in rice plants . Plant Cell Rep . 26 , 2009–2015. 10.1007/s00299-007-0405-9 [ PubMed ] [ CrossRef ] [ Google Scholar ]
- Kapoor D., Singh M. P., Kaur S., Bhardwaj R., Zheng B., Sharma A. (2019). Modulation of the functional components of growth, photosynthesis, and anti-oxidant stress markers in cadmium exposed Brassica juncea L . Plants 8 :260. 10.3390/plants8080260 [ PMC free article ] [ PubMed ] [ CrossRef ] [ Google Scholar ]
- Kappachery S., Baniekal-Hiremath G., Yu J. W., Park S. W. (2015). Effect of over-and under-expression of glyceraldehyde 3-phosphate dehydrogenase on tolerance of plants to water-deficit stress . Plant Cell. Tissue Organ Cult . 121 , 97–107. 10.1007/s11240-014-0684-0 [ CrossRef ] [ Google Scholar ]
- Karaba A., Dixit S., Greco R., Aharoni A., Trijatmiko K. R., Marsch-Martinez N., et al.. (2007). Improvement of water use efficiency in rice by expression of HARDY, an Arabidopsis drought and salt tolerance gene . Proc. Natl. Acad. Sci. U.S.A . 104 , 15270–15275. 10.1073/pnas.0707294104 [ PMC free article ] [ PubMed ] [ CrossRef ] [ Google Scholar ]
- Kaur P., Bali S., Sharma A., Kohli S. K., Vig A. P., Bhardwaj R., et al.. (2019). Cd induced generation of free radical species in Brassica juncea is regulated by supplementation of earthworms in the drilosphere . Sci. Total Environ . 655 , 663–675. 10.1016/j.scitotenv.2018.11.096 [ PubMed ] [ CrossRef ] [ Google Scholar ]
- Kaur R., Yadav P., Sharma A., Kumar Thukral A., Kumar V., Kaur Kohli S., et al.. (2017). Castasterone and citric acid treatment restores photosynthetic attributes in Brassica juncea L. under Cd(II) toxicity . Ecotoxicol. Environ. Saf . 145 , 466–475. 10.1016/j.ecoenv.2017.07.067 [ PubMed ] [ CrossRef ] [ Google Scholar ]
- Khafagy M. A., Arafa A., El-Banna M. F. (2009). Glycinebetaine and ascorbic acid can alleviate the harmful effects of NaCl salinity in sweet pepper . Aust. J. Crop Sci . 3 , 257–267. [ Google Scholar ]
- Khan M. I. R., Khan N. A. (2014). Ethylene reverses photosynthetic inhibition by nickel and zinc in mustard through changes in PS II activity, photosynthetic nitrogen use efficiency, and antioxidant metabolism . Protoplasma 251 , 1007–1019. 10.1007/s00709-014-0610-7 [ PubMed ] [ CrossRef ] [ Google Scholar ]
- Khan M. A., Shirazi M. U., Khan M. A., Mujtaba S. M., Islam E., Mumtaz S., et al. (2009). Role of proline, K/NA ratio and chlorophyll content in salt tolerance of wheat ( Triticum aestivum L.) . Pakistan J. Bot . 41 , 633–638. [ Google Scholar ]
- Khanna K., Jamwal V. L., Sharma A., Gandhi S. G., Ohri P., Bhardwaj R., et al.. (2019). Supplementation with plant growth promoting rhizobacteria (PGPR) alleviates cadmium toxicity in solanum lycopersicum by modulating the expression of secondary metabolites . Chemosphere 230 , 628–639. 10.1016/j.chemosphere.2019.05.072 [ PubMed ] [ CrossRef ] [ Google Scholar ]
- Kidokoro S., Watanabe K., Ohori T., Moriwaki T., Maruyama K., Mizoi J., et al.. (2015). Soybean DREB1/CBF-type transcription factors function in heat and drought as well as cold stress-responsive gene expression . Plant J . 81 , 505–518. 10.1111/tpj.12746 [ PubMed ] [ CrossRef ] [ Google Scholar ]
- Kim K., Portis A. R. (2006). Kinetic analysis of the slow inactivation of Rubisco during catalysis: effects of temperature, O 2 and Mg++ . Photosynth. Res . 87 :195. 10.1007/s11120-005-8386-4 [ PubMed ] [ CrossRef ] [ Google Scholar ]
- Kim T. W., Michniewicz M., Bergmann D. C., Wang Z. Y. (2012). Brassinosteroid regulates stomatal development by GSK3-mediated inhibition of a MAPK pathway . Nature 482 , 419–422. 10.1038/nature10794 [ PMC free article ] [ PubMed ] [ CrossRef ] [ Google Scholar ]
- Kohli S. K., Handa N., Bali S., Khanna K., Arora S., Sharma A., et al.. (2020). Current scenario of Pb toxicity in plants: unraveling plethora of physiological responses . Rev. Environ. Contamin. Toxicol . 249 , 153–197. 10.1007/398_2019_25 [ PubMed ] [ CrossRef ] [ Google Scholar ]
- Kohli S. K., Handa N., Sharma A., Gautam V., Arora S., Bhardwaj R., et al.. (2018). Interaction of 24-epibrassinolide and salicylic acid regulates pigment contents, antioxidative defense responses, and gene expression in Brassica juncea L. seedlings under Pb stress . Environ. Sci. Pollut. Res . 25 , 15159–15173. 10.1007/s11356-018-1742-7 [ PubMed ] [ CrossRef ] [ Google Scholar ]
- Kohli S. K., Handa N., Sharma A., Kumar V., Kaur P., Bhardwaj R. (2017). Synergistic effect of 24-epibrassinolide and salicylic acid on photosynthetic efficiency and gene expression in Brassica juncea L. Under Pb stress . Turkish J. Biol . 41 , 943–953. 10.3906/biy-1707-15 [ PMC free article ] [ PubMed ] [ CrossRef ] [ Google Scholar ]
- Kołodziejczyk I., Posmyk M. M. (2016). Melatonin – a new plant biostimulator? J. Elem . 21 , 1187–1198. 10.5601/jelem.2015.20.3.1012 [ CrossRef ] [ Google Scholar ]
- Komatsu T., Kawaide H., Saito C., Yamagami A., Shimada S., Nakazawa M., et al.. (2010). The chloroplast protein BPG2 functions in brassinosteroid-mediated post-transcriptional accumulation of chloroplast rRNA . Plant J . 61 , 409–422. 10.1111/j.1365-313X.2009.04077.x [ PubMed ] [ CrossRef ] [ Google Scholar ]
- Komayama K., Khatoon M., Takenaka D., Horie J., Yamashita A., Yoshioka M., et al.. (2007). Quality control of photosystem II: cleavage and aggregation of heat-damaged D1 protein in spinach thylakoids . Biochim. Biophys. Acta Bioenerg . 1767 , 838–846. 10.1016/j.bbabio.2007.05.001 [ PubMed ] [ CrossRef ] [ Google Scholar ]
- Kottmann L., Schittenhelm S., Giesemann A. (2014). Suitability of carbon isotope discrimination, ash content and single mineral concentration for the selection of drought-tolerant winter rye . Plant Breed . 133 , 579–587. 10.1111/pbr.12198 [ CrossRef ] [ Google Scholar ]
- Krause G. H., Grube E., Koroleva O. Y., Barth C., Winter K. (2004). Do mature shade leaves of tropical tree seedlings acclimate to high sunlight and UV radiation? Funct. Plant Biol . 31 , 743–756. 10.1071/FP03239 [ PubMed ] [ CrossRef ] [ Google Scholar ]
- Krumova S., Zhiponova M., Dankov K., Velikova V., Balashev K., Andreeva T., et al.. (2013). Brassinosteroids regulate the thylakoid membrane architecture and the photosystem II function . J. Photochem. Photobiol. B Biol . 126 , 97–104. 10.1016/j.jphotobiol.2013.07.008 [ PubMed ] [ CrossRef ] [ Google Scholar ]
- Krupa Z. (1999). Cadmium against higher plant photosynthesis - a variety of effects and where do they possibly come from? Zeitschrift fur Naturforsch . Sect. C J. Biosci . 54 , 723–729. 10.1515/znc-1999-9-1017 [ CrossRef ] [ Google Scholar ]
- Ksas B., Becuwe N., Chevalier A., Havaux M. (2015). Plant tolerance to excess light energy and photooxidative damage relies on plastoquinone biosynthesis . Sci. Rep . 5 :10919. 10.1038/srep10919 [ PMC free article ] [ PubMed ] [ CrossRef ] [ Google Scholar ]
- Kumar S., Singh B. (2009). Effect of water stress on carbon isotope discrimination and Rubisco activity in bread and durum wheat genotypes . Physiol. Mol. Biol. Plants . 15 , 281–286. 10.1007/s12298-009-0032-8 [ PMC free article ] [ PubMed ] [ CrossRef ] [ Google Scholar ]
- Kumar V., Parihar R. D., Sharma A., Bakshi P., Singh Sidhu G. P., Bali A. S., et al.. (2019). Global evaluation of heavy metal content in surface water bodies: a meta-analysis using heavy metal pollution indices and multivariate statistical analyses . Chemosphere . 236 :124364. 10.1016/j.chemosphere.2019.124364 [ PubMed ] [ CrossRef ] [ Google Scholar ]
- Kunihiro S., Hiramatsu T., Kawano T. (2011). Involvement of salicylic acid signal transduction in aluminum-responsive oxidative burst in Arabidopsis thaliana cell suspension culture . Plant Signal. Behav . 6 , 611–616. 10.4161/psb.6.5.14895 [ PMC free article ] [ PubMed ] [ CrossRef ] [ Google Scholar ]
- Kurepin L. V., Dahal K. P., Savitch L. V., Singh J., Bode R., Ivanov A. G., et al.. (2013). Role of CBFs as integrators of chloroplast redox, phytochrome and plant hormone signaling during cold acclimation . Int. J. Mol. Sci . 14 , 12729–12763. 10.3390/ijms140612729 [ PMC free article ] [ PubMed ] [ CrossRef ] [ Google Scholar ]
- Laing W. A., Ogren W. L., Hageman R. H. (1974). Regulation of soybean net photosynthetic CO 2 fixation by the interaction of CO 2 , O 2 , and ribulose 1,5-diphosphate carboxylase . Plant Physiol . 54 , 678–685. 10.1104/pp.54.5.678 [ PMC free article ] [ PubMed ] [ CrossRef ] [ Google Scholar ]
- Laureau C., De Paepe R., Latouche G., Moreno-Chacón M., Finazzi G., Kuntz M., et al.. (2013). Plastid terminal oxidase (PTOX) has the potential to act as a safety valve for excess excitation energy in the alpine plant species Ranunculus glacialis L . Plant Cell Environ . 36 , 1296–1310. 10.1111/pce.12059 [ PubMed ] [ CrossRef ] [ Google Scholar ]
- Lawlor D. W. (2009). Musings about the effects of environment on photosynthesis . Ann. Bot . 103 , 543–549. 10.1093/aob/mcn256 [ PMC free article ] [ PubMed ] [ CrossRef ] [ Google Scholar ]
- Lee Y. P., Kim S. H., Bang J. W., Lee H. S., Kwak S. S., Kwon S. Y. (2007). Enhanced tolerance to oxidative stress in transgenic tobacco plants expressing three antioxidant enzymes in chloroplasts . Plant Cell Rep . 26 , 591–598. 10.1007/s00299-006-0253-z [ PubMed ] [ CrossRef ] [ Google Scholar ]
- Li C., Tan D.-X., Liang D., Chang C., Jia D., Ma F. (2014). Melatonin mediates the regulation of ABA metabolism, free-radical scavenging, and stomatal behaviour in two Malus species under drought stress . J. Exp. Bot . 66 , 669–680. 10.1093/jxb/eru476 [ PubMed ] [ CrossRef ] [ Google Scholar ]
- Li H., Chang J., Chen H., Wang Z., Gu X., Wei C., et al.. (2017). Exogenous melatonin confers salt stress tolerance to watermelon by improving photosynthesis and redox homeostasis . Front. Plant Sci . 8 :295. 10.3389/fpls.2017.00295 [ PMC free article ] [ PubMed ] [ CrossRef ] [ Google Scholar ]
- Li M., Ahammed G. J., Li C., Bao X., Yu J., Huang C., et al.. (2016). Brassinosteroid ameliorates zinc oxide nanoparticles-induced oxidative stress by improving antioxidant potential and redox homeostasis in tomato seedling . Front. Plant Sci . 7 :615. 10.3389/fpls.2016.00615 [ PMC free article ] [ PubMed ] [ CrossRef ] [ Google Scholar ]
- Li M., Liu H., Li L., Yi X., Zhu X. (2007). Carbon isotope composition of plants along altitudinal gradient and its relationship to environmental factors on the Qinghai-Tibet Plateau . Polish J. Ecol . 55 , 67–68. [ Google Scholar ]
- Li R., He J., Xie H., Wang W., Bose S. K., Sun Y., et al.. (2019). Effects of chitosan nanoparticles on seed germination and seedling growth of wheat ( Triticum aestivum L.) . Int. J. Biol. Macromol . 126 , 91–100. 10.1016/j.ijbiomac.2018.12.118 [ PubMed ] [ CrossRef ] [ Google Scholar ]
- Li T. X. Y. Z., Zhang Y., Liu H., Wu Y. T., Li W., Bin, Zhang H. X. (2010). Stable expression of arabidopsis vacuolar Na+/H+ antiporter gene AtNHX1, and salt tolerance in transgenic soybean for over six generations . Chinese Sci. Bull . 55 , 1127–1134. 10.1007/s11434-010-0092-8 [ CrossRef ] [ Google Scholar ]
- Li Z., Wakao S., Fischer B. B., Niyogi K. K. (2009). Sensing and responding to excess light . Annu. Rev. Plant Biol . 60 , 239–60. 10.1146/annurev.arplant.58.032806.103844 [ PubMed ] [ CrossRef ] [ Google Scholar ]
- Li Z., Yu J., Peng Y., Huang B. (2017). Metabolic pathways regulated by abscisic acid, salicylic acid and γ-aminobutyric acid in association with improved drought tolerance in creeping bentgrass ( Agrostis stolonifera ) . Physiol. Plant . 159 , 42–58. 10.1111/ppl.12483 [ PubMed ] [ CrossRef ] [ Google Scholar ]
- Liang C., Zheng G., Li W., Wang Y., Hu B., Wang H., et al.. (2015). Melatonin delays leaf senescence and enhances salt stress tolerance in rice . J. Pineal Res . 59 , 91–101. 10.1111/jpi.12243 [ PubMed ] [ CrossRef ] [ Google Scholar ]
- Liang D., Gao F., Ni Z., Lin L., Deng Q., Tang Y., et al.. (2018). Melatonin improves heat tolerance in kiwifruit seedlings through promoting antioxidant enzymatic activity and glutathione S-transferase transcription . Molecules 23 :584. 10.3390/molecules23030584 [ PMC free article ] [ PubMed ] [ CrossRef ] [ Google Scholar ]
- Lípová L., Krchnák P., Komenda J., Ilík P. (2010). Heat-induced disassembly and degradation of chlorophyll-containing protein complexes in vivo . Biochim. Biophys. Acta - Bioenerg . 1797 , 63–70. 10.1016/j.bbabio.2009.08.001 [ PubMed ] [ CrossRef ] [ Google Scholar ]
- Liu Q., hua, Wu X., Chen B., cong, Ma J., qing, Gao J. (2014). Effects of low light on agronomic and physiological characteristics of rice including grain yield and quality . Rice Sci . 21 , 243–251. 10.1016/S1672-6308(13)60192-4 [ CrossRef ] [ Google Scholar ]
- Liu R., Xu Y. H., Jiang S. C., Lu K., Lu Y. F., Feng X. J., et al.. (2013). Light-harvesting chlorophyll a/b-binding proteins, positively involved in abscisic acid signalling, require a transcription repressor, WRKY40, to balance their function . J. Exp. Bot . 64 , 5443–5456. 10.1093/jxb/ert307 [ PMC free article ] [ PubMed ] [ CrossRef ] [ Google Scholar ]
- Macková J., VaŠková M., Macek P., Hronková M., Schreiber L., Šantruček J. (2013). Plant response to drought stress simulated by ABA application: changes in chemical composition of cuticular waxes . Environ. Exp. Bot . 86 , 70–75. 10.1016/j.envexpbot.2010.06.005 [ CrossRef ] [ Google Scholar ]
- Makino A. (2011). Photosynthesis, grain yield, and nitrogen utilization in rice and wheat . Plant Physiol . 155 , 125–129. 10.1104/pp.110.165076 [ PMC free article ] [ PubMed ] [ CrossRef ] [ Google Scholar ]
- Manna S. (2015). An overview of pentatricopeptide repeat proteins and their applications . Biochimie 113 , 93–99. 10.1016/j.biochi.2015.04.004 [ PubMed ] [ CrossRef ] [ Google Scholar ]
- Mashiguchi K., Sasaki E., Shimada Y., Nagae M., Ueno K., Nakano T., et al.. (2009). Feedback-regulation of strigolactone biosynthetic genes and strigolactone-regulated genes in Arabidopsis . Biosci. Biotechnol. Biochem . 73 , 2460–2465. 10.1271/bbb.90443 [ PubMed ] [ CrossRef ] [ Google Scholar ]
- Maslenkova L. T., Zanev Y., Popova L. P. (1989). Effect of abscisic acid on the photosynthetic oxygen evolution in barley chloroplasts . Photosynth. Res . 21 , 45–50. 10.1007/BF00047174 [ PubMed ] [ CrossRef ] [ Google Scholar ]
- Mathur S., Agrawal D., Jajoo A. (2014). Photosynthesis: response to high temperature stress . J. Photochem. Photobiol. B Biol . 137 , 116–126. 10.1016/j.jphotobiol.2014.01.010 [ PubMed ] [ CrossRef ] [ Google Scholar ]
- Maxwell B. B., Andersson C. R., Poole D. S., Kay S. A., Chory J. (2003). HY5, circadian clock-associated 1, and a cis-element, DET1 dark response element, mediate DET1 regulation of chlorophyll a/b-binding protein 2 expression . Plant Physiol . 133 , 1565–1577. 10.1104/pp.103.025114 [ PMC free article ] [ PubMed ] [ CrossRef ] [ Google Scholar ]
- Mayzlish-Gati E., LekKala S. P., Resnick N., Wininger S., Bhattacharya C., Lemcoff J. H., et al. (2010). Strigolactones are positive regulators of light-harvesting genes in tomato . J. Exp. Bot . 61 , 3129–3136. 10.1093/jxb/erq138 [ PMC free article ] [ PubMed ] [ CrossRef ] [ Google Scholar ]
- Medrano H., Escalona J. M., Bota J., Gulías J., Flexas J. (2002). Regulation of photosynthesis of C3 plants in response to progressive drought: stomatal conductance as a reference parameter . Ann. Bot . 89 , 895–905. 10.1093/aob/mcf079 [ PMC free article ] [ PubMed ] [ CrossRef ] [ Google Scholar ]
- Meganid A. S., Al-Zahrani H. S., Selim E. M. (2015). Effect of humic acid application on growth and chlorophyll contents of common bean plants ( Phaseolus vulgaris L.) under salinity stress conditions . Int. J. Innov. Res. Sci. Eng. Technol . 4 , 2651–2660. 10.15680/ijirset.2015.0405001 [ CrossRef ] [ Google Scholar ]
- Meng J. F., Xu T. F., Wang Z. Z., Fang Y. L., Xi Z. M., Zhang Z. W. (2014). The ameliorative effects of exogenous melatonin on grape cuttings under water-deficient stress: antioxidant metabolites, leaf anatomy, and chloroplast morphology . J. Pineal Res . 57 , 200–212. 10.1111/jpi.12159 [ PubMed ] [ CrossRef ] [ Google Scholar ]
- Merah O., Evon P., Monneveux P. (2018). Participation of green organs to grain filling in triticum turgidum var durum grown under mediterranean conditions . Int. J. Mol. Sci . 19 :56. 10.3390/ijms19010056 [ PMC free article ] [ PubMed ] [ CrossRef ] [ Google Scholar ]
- Miller G., Suzuki N., Ciftci-Yilmaz S., Mittler R. (2010). Reactive oxygen species homeostasis and signalling during drought and salinity stresses . Plant Cell Environ . 33 , 453–467. 10.1111/j.1365-3040.2009.02041.x [ PubMed ] [ CrossRef ] [ Google Scholar ]
- Mittal S., Kumari N., Sharma V. (2012). Differential response of salt stress on Brassica juncea : photosynthetic performance, pigment, proline, D1 and antioxidant enzymes . Plant Physiol. Biochem . 54 , 17–26. 10.1016/j.plaphy.2012.02.003 [ PubMed ] [ CrossRef ] [ Google Scholar ]
- Mittler R., Finka A., Goloubinoff P. (2012). How do plants feel the heat? Trends Biochem. Sci . 17 , 118–125. 10.1016/j.tibs.2011.11.007 [ PubMed ] [ CrossRef ] [ Google Scholar ]
- Mittler R., Vanderauwera S., Gollery M., Van Breusegem F. (2004). Reactive oxygen gene network of plants . Trends Plant Sci . 9 , 490–498. 10.1016/j.tplants.2004.08.009 [ PubMed ] [ CrossRef ] [ Google Scholar ]
- Mohanty S., Grimm B., Tripathy B. C. (2006). Light and dark modulation of chlorophyll biosynthetic genes in response to temperature . Planta 224 , 692–699. 10.1007/s00425-006-0248-6 [ PubMed ] [ CrossRef ] [ Google Scholar ]
- Molas J. (2002). Changes of chloroplast ultrastructure and total chlorophyll concentration in cabbage leaves caused by excess of organic Ni(II) complexes . Environ. Exp. Bot . 47 , 115–126. 10.1016/S0098-8472(01)00116-2 [ CrossRef ] [ Google Scholar ]
- Monirifar H., Barghi M. (2009). Identification and selection for salt tolerance in alfalfa ( Medicago sativa L.) ecotypes via physiological traits . Not. Sci. Biol . 1 , 63–66. 10.15835/nsb113498 [ CrossRef ] [ Google Scholar ]
- Mu X., Chen Q., Chen F., Yuan L., Mi G. (2016). Within-leaf nitrogen allocation in adaptation to low nitrogen supply in maize during grain-filling stage . Front. Plant Sci . 7 :699. 10.3389/fpls.2016.00699 [ PMC free article ] [ PubMed ] [ CrossRef ] [ Google Scholar ]
- Mu X., Chen Q., Chen F., Yuan L., Mi G. (2017). A RNA-seq analysis of the response of photosynthetic system to low nitrogen supply in maize leaf . Int. J. Mol. Sci . 18 :2624. 10.3390/ijms18122624 [ PMC free article ] [ PubMed ] [ CrossRef ] [ Google Scholar ]
- Mukhtar Ahmed K. B., Khan M. M. A., Siddiqui H., Jahan A. (2020). Chitosan and its oligosaccharides, a promising option for sustainable crop production- a review . Carbohydr. Polym . 227 :115331. 10.1016/j.carbpol.2019.115331 [ PubMed ] [ CrossRef ] [ Google Scholar ]
- Mumm P., Wolf T., Fromm J., Roelfsema M. R. G., Marten I. (2011). Cell type-specific regulation of ion channels within the maize stomatal complex . Plant Cell Physiol . 52 , 1365–1375. 10.1093/pcp/pcr082 [ PubMed ] [ CrossRef ] [ Google Scholar ]
- Munné-Bosch S., Peñuelas J. (2003). Photo- and antioxidative protection, and a role for salicylic acid during drought and recovery in field-grown Phillyrea angustifolia plants . Planta 217 , 758–766. 10.1007/s00425-003-1037-0 [ PubMed ] [ CrossRef ] [ Google Scholar ]
- Muranaka S., Shimizu K., Kato M. (2002). Ionic and osmotic effects of salinity on single-leaf photosynthesis in two wheat cultivars with different drought tolerance . Photosynthetica 40 , 201–207. 10.1023/A:1021337522431 [ CrossRef ] [ Google Scholar ]
- Najafpour M. M., Ghobadi M. Z., Larkum A. W., Shen J. R., Allakhverdiev S. I. (2015). The biological water-oxidizing complex at the nano-bio interface . Trends Plant Sci . 20 , 559–568. 10.1016/j.tplants.2015.06.005 [ PubMed ] [ CrossRef ] [ Google Scholar ]
- Najeeb U., Jilani G., Ali S., Sarwar M., Xu L., Zhou W. (2011). Insights into cadmium induced physiological and ultra-structural disorders in Juncus effusus L. and its remediation through exogenous citric acid . J. Hazard. Mater . 186 , 565–574. 10.1016/j.jhazmat.2010.11.037 [ PubMed ] [ CrossRef ] [ Google Scholar ]
- Nath K., Jajoo A., Poudyal R. S., Timilsina R., Park Y. S., Aro E. M., et al.. (2013). Towards a critical understanding of the photosystem II repair mechanism and its regulation during stress conditions . FEBS Lett . 587 , 3372–3381. 10.1016/j.febslet.2013.09.015 [ PubMed ] [ CrossRef ] [ Google Scholar ]
- Nawaz M. A., Huang Y., Bie Z., Ahmed W., Reiter R. J., Niu M., et al.. (2016). Melatonin: current status and future perspectives in plant science . Front. Plant Sci . 6 :1230. 10.3389/fpls.2015.01230 [ PMC free article ] [ PubMed ] [ CrossRef ] [ Google Scholar ]
- Nawaz M. A., Jiao Y., Chen C., Shireen F., Zheng Z., Imtiaz M., et al.. (2018). Melatonin pretreatment improves vanadium stress tolerance of watermelon seedlings by reducing vanadium concentration in the leaves and regulating melatonin biosynthesis and antioxidant-related gene expression . J. Plant Physiol . 220 , 115–127. 10.1016/j.jplph.2017.11.003 [ PubMed ] [ CrossRef ] [ Google Scholar ]
- Nguyen C. V., Vrebalov J. T., Gapper N. E., Zheng Y., Zhong S., Fei Z., et al.. (2014). Tomato GOLDEN2-LIKE transcription factors reveal molecular gradients that function during fruit development and ripening . Plant Cell 26 , 585–601. 10.1105/tpc.113.118794 [ PMC free article ] [ PubMed ] [ CrossRef ] [ Google Scholar ]
- Nguyen H. C., Lin K. H., Hsiung T. C., Huang M. Y., Yang C. M., Weng J. H., et al. (2018). Biochemical and physiological characteristics of photosynthesis in plants of two calathea species . Int. J. Mol. Sci . 19 :704 10.3390/ijms19030704 [ PMC free article ] [ PubMed ] [ CrossRef ] [ Google Scholar ]
- Nishiyama Y., Murata N. (2014). Revised scheme for the mechanism of photoinhibition and its application to enhance the abiotic stress tolerance of the photosynthetic machinery . Appl. Microbiol. Biotechnol . 98 , 8777–8796. 10.1007/s00253-014-6020-0 [ PubMed ] [ CrossRef ] [ Google Scholar ]
- Nishiyama R., Watanabe Y., Fujita Y., Le D. T., Kojima M., Werner T., et al.. (2011). Analysis of cytokinin mutants and regulation of cytokinin metabolic genes reveals important regulatory roles of cytokinins in drought, salt and abscisic acid responses, and abscisic acid biosynthesis . Plant Cell . 23 , 2169–2183. 10.1105/tpc.111.087395 [ PMC free article ] [ PubMed ] [ CrossRef ] [ Google Scholar ]
- Noctor G., Mhamdi A., Foyer C. H. (2014). The roles of reactive oxygen metabolism in drought: not so cut and dried . Plant Physiol . 164 , 1636–1648. 10.1104/pp.113.233478 [ PMC free article ] [ PubMed ] [ CrossRef ] [ Google Scholar ]
- Noreen Z., Ashraf M., Akram N. A. (2010). Salt-induced modulation in some key gas exchange characteristics and ionic relations in pea ( Pisum sativum L.) and their use as selection criteria . Crop Pasture Sci . 61 , 396–378. 10.1071/CP09255 [ CrossRef ] [ Google Scholar ]
- Nouri M. Z., Moumeni A., Komatsu S. (2015). Abiotic stresses: insight into gene regulation and protein expression in photosynthetic pathways of plants . Int. J. Mol. Sci . 16 , 20392–20416. 10.3390/ijms160920392 [ PMC free article ] [ PubMed ] [ CrossRef ] [ Google Scholar ]
- Oh M. H., Sun J., Oh D. H., Zielinski R. E., Clouse S. D., Huber S. C. (2011). Enhancing arabidopsis leaf growth by engineering the brassinosteroid insensitive1 receptor kinase . Plant Physiol . 157 , 120–131. 10.1104/pp.111.182741 [ PMC free article ] [ PubMed ] [ CrossRef ] [ Google Scholar ]
- Oh M. H., Wang X., Wu X., Zhao Y., Clouse S. D., Huber S. C. (2010). Autophosphorylation of Tyr-610 in the receptor kinase BAK1 plays a role in brassinosteroid signaling and basal defense gene expression . Proc. Natl. Acad. Sci. U.S.A . 107 , 17827–17832. 10.1073/pnas.0915064107 [ PMC free article ] [ PubMed ] [ CrossRef ] [ Google Scholar ] Retracted
- Omoto E., Taniguchi M., Miyake H. (2010). Effects of salinity stress on the structure of bundle sheath and mesophyll chloroplasts in NAD-malic enzyme and PCK type C4 plants . Plant Prod. Sci . 13 , 169–176. 10.1626/pps.13.169 [ CrossRef ] [ Google Scholar ]
- Ouzounidou G., Ilias I. (2005). Hormone-induced protection of sunflower photosynthetic apparatus against copper toxicity . Biol. Plant . 49 :223 10.1007/s10535-005-3228-y [ CrossRef ] [ Google Scholar ]
- Pan J., Guo B. (2016). Effects of light intensity on the growth, photosynthetic characteristics, and flavonoid content of Epimedium pseudowushanense B.L.Guo . Molecules 21 :1475. 10.3390/molecules21111475 [ PMC free article ] [ PubMed ] [ CrossRef ] [ Google Scholar ]
- Pan J., Lin S., Woodbury N. W. (2012). Bacteriochlorophyll excited-state quenching pathways in bacterial reaction centers with the primary donor oxidized . J. Phys. Chem. B . 116 , 2014–2022. 10.1021/jp212441b [ PubMed ] [ CrossRef ] [ Google Scholar ]
- Parmar P., Kumari N., Sharma V. (2013). Structural and functional alterations in photosynthetic apparatus of plants under cadmium stress . Bot. Stud . 54 :45. 10.1186/1999-3110-54-45 [ PMC free article ] [ PubMed ] [ CrossRef ] [ Google Scholar ]
- Patra B., Ray S., Richter A., Majumder A. L. (2010). Enhanced salt tolerance of transgenic tobacco plants by co-expression of PcINO1 and McIMT1 is accompanied by increased level of myo-inositol and methylated inositol . Protoplasma 245 , 143–52. 10.1007/s00709-010-0163-3 [ PubMed ] [ CrossRef ] [ Google Scholar ]
- Pätsikkä E., Kairavuo M., Šeršen F., Aro E. M., Tyystjärvi E. (2002). Excess copper predisposes photosystem II to photoinhibition in vivo by outcompeting iron and causing decrease in leaf chlorophyll . Plant Physiol . 129 , 1359–1367. 10.1104/pp.004788 [ PMC free article ] [ PubMed ] [ CrossRef ] [ Google Scholar ]
- Paunov M., Koleva L., Vassilev A., Vangronsveld J., Goltsev V. (2018). Effects of different metals on photosynthesis: cadmium and zinc affect chlorophyll fluorescence in durum wheat . Int. J. Mol. Sci . 19 :787. 10.3390/ijms19030787 [ PMC free article ] [ PubMed ] [ CrossRef ] [ Google Scholar ]
- Pedhadiya M. D., Vaishnav P. P., Singh Y. D. (1987). Development of photosynthetic electron transport reactions under the influence of phytohormones and nitrate nutrition in greening cucumber cotyledons . Photosynth. Res . 13 , 159–165. 10.1007/BF00035238 [ PubMed ] [ CrossRef ] [ Google Scholar ]
- Pego J. V., Kortstee A. J., Huijser C., Smeekens S. C. M. (2000). Photosynthesis, sugars and the regulation of gene expression . J. Exp. Bot . 51 , 407–416. 10.1093/jexbot/51.suppl_1.407 [ PubMed ] [ CrossRef ] [ Google Scholar ]
- Pego J. V., Weisbeek P. J., Smeekens S. C. M. (1999). Mannose inhibits Arabidopsis germination via a hexokinase-mediated step . Plant Physiol . 119 , 1017–1123. 10.1104/pp.119.3.1017 [ PMC free article ] [ PubMed ] [ CrossRef ] [ Google Scholar ]
- Pérez P., Alonso A., Zita G., Morcuende R., Martínez-Carrasco R. (2011). Down-regulation of Rubisco activity under combined increases of CO 2 and temperature minimized by changes in Rubisco kcat in wheat . Plant Growth Regul . 65 , 439–447. 10.1007/s10725-011-9613-y [ CrossRef ] [ Google Scholar ]
- Perveen S., Shahbaz M., Ashraf M. (2010). Regulation in gas exchange and quantum yield of photosystem II (PSII) in salt-stressed and non-stressed wheat plants raised from seed treated with triacontanol . Pakistan J. Bot . 42 , 3073–3081. [ Google Scholar ]
- Petrozza A., Santaniello A., Summerer S., Di Tommaso G., Di Tommaso D., Paparelli E., et al. (2014). Physiological responses to Megafol® treatments in tomato plants under drought stress: a phenomic and molecular approach . Sci. Hortic . 174 , 185–192. 10.1016/j.scienta.2014.05.023 [ CrossRef ] [ Google Scholar ]
- Pinheiro H. A., Silva J. V., Endres L., Ferreira V. M., de Câmara C. A., Cabral F. F., et al. (2008). Leaf gas exchange, chloroplastic pigments and dry matter accumulation in castor bean (Ricinus communis L) seedlings subjected to salt stress conditions . Ind. Crops Prod . 27 , 385–392. 10.1016/j.indcrop.2007.10.003 [ CrossRef ] [ Google Scholar ]
- Pirasteh-Anosheh H., Saed-Moucheshi A., Pakniyat H., Pessarakli M. (2016). Stomatal responses to drought stress , in Water Stress and Crop Plants: A Sustainable Approach . ed Ahmad P. (John Wiley and Sons, Ltd.) 24–40. 10.1002/9781119054450.ch3 [ CrossRef ] [ Google Scholar ]
- Pirzad A., Shakiba M. R., Zehtab-Salmasi S., Mohammadi S. A., Darvishzadeh R., Samadi A. (2011). Effect of water stress on leaf relative water content, chlorophyll, proline and soluble carbohydrates in Matricaria chamomilla L . J. Med. Plants Res . 5 , 2483–2488. 10.5897/JMPR.9000503 [ CrossRef ] [ Google Scholar ]
- Posmyk M. M., Kuran H., Marciniak K., Janas K. M. (2008). Presowing seed treatment with melatonin protects red cabbage seedlings against toxic copper ion concentrations . J. Pineal Res . 45 , 24–31. 10.1111/j.1600-079X.2007.00552.x [ PubMed ] [ CrossRef ] [ Google Scholar ]
- Pospíšil P. (2014). The role of metals in production and scavenging of reactive oxygen species in photosystem II . Plant Cell Physiol . 55 , 1224–1232. 10.1093/pcp/pcu053 [ PubMed ] [ CrossRef ] [ Google Scholar ]
- Pusnik M., Small I., Read L. K., Fabbro T., Schneider A. (2007). Pentatricopeptide repeat proteins in trypanosoma brucei function in mitochondrial ribosomes . Mol. Cell. Biol . 27 , 6876–6888. 10.1128/mcb.00708-07 [ PMC free article ] [ PubMed ] [ CrossRef ] [ Google Scholar ]
- Raines C. A. (2011). Increasing photosynthetic carbon assimilation in C3 plants to improve crop yield: current and future strategies . Plant Physiol . 155 , 36–42. 10.1104/pp.110.168559 [ PMC free article ] [ PubMed ] [ CrossRef ] [ Google Scholar ]
- Raja V., Majeed U., Kang H., Andrabi K. I., John R. (2017). Abiotic stress: interplay between ROS, hormones and MAPKs . Environ. Exp. Bot . 137 , 142–157. 10.1016/j.envexpbot.2017.02.010 [ CrossRef ] [ Google Scholar ]
- Rascio N., Navari-Izzo F. (2011). Heavy metal hyperaccumulating plants: how and why do they do it? And what makes them so interesting? Plant Sci . 180 , 169–181. 10.1016/j.plantsci.2010.08.016 [ PubMed ] [ CrossRef ] [ Google Scholar ]
- Raza S. H., Athar H. U. R., Ashraf M. (2006). Influence of exogenously applied glycinebetaine on the photosynthetic capacity of two differently adapted wheat cultivars under salt stress . Pakistan J. Bot . 38 , 341–351. [ Google Scholar ]
- Reda F., Mandoura H. M. H. (2011). Response of enzymes activities, photosynthetic pigments, proline to low or high temperature stressed wheat plant ( Triticum Aestivum L.) in the presence or absence of exogenous proline or cysteine . Int. J. Acad. Res . 3 , 108–115. [ Google Scholar ]
- Reiter R. J., Tan D. X., Zhou Z., Cruz M. H. C., Fuentes-Broto L., Galano A. (2015). Phytomelatonin: assisting plants to survive and thrive . Molecules 20 , 7396–7437. 10.3390/molecules20047396 [ PMC free article ] [ PubMed ] [ CrossRef ] [ Google Scholar ]
- Rexroth S., Mullineaux C. W., Ellinger D., Sendtko E., Rögner M., Koenig F. (2011). The plasma membrane of the cyanobacterium gloeobacter violaceus contains segregated bioenergetic domains . Plant Cell . 23 , 2379–2390. 10.1105/tpc.111.085779 [ PMC free article ] [ PubMed ] [ CrossRef ] [ Google Scholar ]
- Ristic Z., Bukovnik U., Momčilović I., Fu J., Vara Prasad P. V. (2008). Heat-induced accumulation of chloroplast protein synthesis elongation factor, EF-Tu, in winter wheat . J. Plant Physiol . 165 , 192–202. 10.1016/j.jplph.2007.03.003 [ PubMed ] [ CrossRef ] [ Google Scholar ]
- Rivero R. M., Gimeno J., Van Deynze A., Walia H., Blumwald E. (2010). Enhanced cytokinin synthesis in tobacco plants expressing PSARK::IPT prevents the degradation of photosynthetic protein complexes during drought . Plant Cell Physiol . 51 , 1929–1941. 10.1093/pcp/pcq143 [ PubMed ] [ CrossRef ] [ Google Scholar ]
- Rivero R. M., Kojima M., Gepstein A., Sakakibara H., Mittler R., Gepstein S., et al.. (2007). Delayed leaf senescence induces extreme drought tolerance in a flowering plant . Proc. Natl. Acad. Sci. U.S.A . 10.1073/pnas.0709453104 [ PMC free article ] [ PubMed ] [ CrossRef ] [ Google Scholar ]
- Romero L., Belakbir A., Ragala L., Ruiz J. M. (1997). Response of plant yield and leaf pigments to saline conditions: effectiveness of different rootstocks in melon plants ( Cucumis melo L.) . Soil Sci. Plant Nutr . 43 , 855–862. 10.1080/00380768.1997.10414652 [ CrossRef ] [ Google Scholar ]
- Rothová O., Holá D., Kočová M., Tumová L., Hnilička F., Hniličková H., et al.. (2014). 24-Epibrassinolide and 20-hydroxyecdysone affect photosynthesis differently in maize and spinach . Steroids 85 , 44–57. 10.1016/j.steroids.2014.04.006 [ PubMed ] [ CrossRef ] [ Google Scholar ]
- Saa S., Del Rio A. O., Castro S., Brown P. H. (2015). Foliar application of microbial and plant based biostimulants increases growth and potassium uptake in almond ( Prunus dulcis [Mill.] D. A. Webb) . Front. Plant Sci . 6 :87. 10.3389/fpls.2015.00087 [ PMC free article ] [ PubMed ] [ CrossRef ] [ Google Scholar ]
- Sabir P., Ashraf M., Hussain M., Jamil A. (2009). Relationship of photosynthetic pigments and water relations with salt tolerance of proso millet ( Panicum Miliaceum L.) accessions . Pakistan J. Bot . 41 , 2957–2964. [ Google Scholar ]
- Sade N., Gebretsadik M., Seligmann R., Schwartz A., Wallach R., Moshelion M. (2010). The role of tobacco aquaporin1 in improving water use efficiency, hydraulic conductivity, and yield production under salt stress . Plant Physiol . 152 , 245–254. 10.1104/pp.109.145854 [ PMC free article ] [ PubMed ] [ CrossRef ] [ Google Scholar ]
- Saibo N. J. M., Lourenço T., Oliveira M. M. (2009). Transcription factors and regulation of photosynthetic and related metabolism under environmental stresses . Ann. Bot . 103 , 609–623. 10.1093/aob/mcn227 [ PMC free article ] [ PubMed ] [ CrossRef ] [ Google Scholar ]
- Salvucci M. E., Werneke J. M., Ogren W. L., Portis A. R. (1987). Purification and species distribution of rubisco activase . Plant Physiol . 84 , 930–936. 10.1104/pp.84.3.930 [ PMC free article ] [ PubMed ] [ CrossRef ] [ Google Scholar ]
- Santos C. V. (2004). Regulation of chlorophyll biosynthesis and degradation by salt stress in sunflower leaves . Sci. Hortic . 103 , 93–99. 10.1016/j.scienta.2004.04.009 [ CrossRef ] [ Google Scholar ]
- Sato R., Ito H., Tanaka A. (2015). Chlorophyll b degradation by chlorophyll b reductase under high-light conditions . Photosynth. Res . 126 , 249–259. 10.1007/s11120-015-0145-6 [ PubMed ] [ CrossRef ] [ Google Scholar ]
- Schaeffer H. J., Forsthoefel N. R., Cushman J. C. (1995). Identification of enhancer and silencer regions involved in salt-responsive expression of Crassulacean acid metabolism (CAM) genes in the facultative halophyte Mesembryanthemum crystallinum . Plant Mol. Biol . 28 , 205–218. 10.1007/BF00020241 [ PubMed ] [ CrossRef ] [ Google Scholar ]
- Schmutz J., Cannon S. B., Schlueter J., Ma J., Mitros T., Nelson W., et al.. (2010). Genome sequence of the palaeopolyploid soybean . Nature . 463 , 178–183. 10.1038/nature08670 [ PubMed ] [ CrossRef ] [ Google Scholar ]
- Schramm F., Larkindale J., Kiehlmann E., Ganguli A., Englich G., Vierling E., et al.. (2008). A cascade of transcription factor DREB2A and heat stress transcription factor HsfA3 regulates the heat stress response of Arabidopsis . Plant J . 53 , 264–274. 10.1111/j.1365-313X.2007.03334.x [ PubMed ] [ CrossRef ] [ Google Scholar ]
- Semerci A., Cicek N., Karahan F. A., Ozyurek E., Kasko Arici Y., Ekmekci Y. (2017). Some growth and chlorophyll fluorescence parameters of black and hybrid poplar clones under water stress . Turkish J. Agric. For . 41 , 348–356. 10.3906/tar-1703-60 [ CrossRef ] [ Google Scholar ]
- Shahzad B., Mughal M. N., Tanveer M., Gupta D., Abbas G. (2017). Is lithium biologically an important or toxic element to living organisms? An overview . Environ. Sci. Pollut. Res . 24 , 103–115. 10.1007/s11356-016-7898-0 [ PubMed ] [ CrossRef ] [ Google Scholar ]
- Shahzad B., Tanveer M., Che Z., Rehman A., Cheema S. A., Sharma A., et al.. (2018a). Role of 24-epibrassinolide (EBL) in mediating heavy metal and pesticide induced oxidative stress in plants: a review . Ecotoxicol. Environ. Saf . 147 , 935–944. 10.1016/j.ecoenv.2017.09.066 [ PubMed ] [ CrossRef ] [ Google Scholar ]
- Shahzad B., Tanveer M., Hassan W., Shah A. N., Anjum S. A., Cheema S. A., et al.. (2016). Lithium toxicity in plants: reasons, mechanisms and remediation possibilities – a review . Plant Physiol. Biochem . 107 , 104–115. 10.1016/j.plaphy.2016.05.034 [ PubMed ] [ CrossRef ] [ Google Scholar ]
- Shahzad B., Tanveer M., Rehman A., Cheema S. A., Fahad S., Rehman S., et al.. (2018b). Nickel; whether toxic or essential for plants and environment - a review . Plant Physiol. Biochem . 132 , 641–651. 10.1016/j.plaphy.2018.10.014 [ PubMed ] [ CrossRef ] [ Google Scholar ]
- Sharif R., Mujtaba M., Rahman M. U., Shalmani A., Ahmad H., Anwar T., et al.. (2018). The multifunctional role of chitosan in horticultural crops; a review . Molecules 23 :872. 10.3390/molecules23040872 [ PMC free article ] [ PubMed ] [ CrossRef ] [ Google Scholar ]
- Sharkey T. D. (2005). Effects of moderate heat stress on photosynthesis: importance of thylakoid reactions, rubisco deactivation, reactive oxygen species, and thermotolerance provided by isoprene . Plant Cell Environ . 28 , 269–277. 10.1111/j.1365-3040.2005.01324.x [ CrossRef ] [ Google Scholar ]
- Sharkey T. D., Zhang R. (2010). High temperature effects on electron and proton circuits of photosynthesis . J. Integr. Plant Biol . 52 , 712–722. 10.1111/j.1744-7909.2010.00975.x [ PubMed ] [ CrossRef ] [ Google Scholar ]
- Sharma A., Kumar V., Shahzad B., Ramakrishnan M., Singh Sidhu G. P., Bali A. S., et al. (2020). Photosynthetic response of plants under different abiotic stresses: a review . J. Plant Growth Regul . 39 , 509–531. 10.1007/s00344-019-10018-x [ CrossRef ] [ Google Scholar ]
- Sharma A., Kumar V., Singh R., Thukral A. K., Bhardwaj R. (2016a). Effect of seed pre-soaking with 24-epibrassinolide on growth and photosynthetic parameters of Brassica juncea L. in imidacloprid soil . Ecotoxicol. Environ. Saf . 133 , 195–201. 10.1016/j.ecoenv.2016.07.008 [ PubMed ] [ CrossRef ] [ Google Scholar ]
- Sharma A., Shahzad B., Kumar V., Kohli S. K., Sidhu G. P. S., Bali A. S., et al.. (2019). Phytohormones regulate accumulation of osmolytes under abiotic stress . Biomolecules 9 :285. 10.3390/biom9070285 [ PMC free article ] [ PubMed ] [ CrossRef ] [ Google Scholar ]
- Sharma A., Thakur S., Kumar V., Kanwar M. K., Kesavan A. K., Thukral A. K., et al.. (2016b). Pre-sowing seed treatment with 24-epibrassinolide ameliorates pesticide stress in Brassica juncea L. through the modulation of stress markers . Front. Plant Sci . 7 :1569. 10.3389/fpls.2016.01569 [ PMC free article ] [ PubMed ] [ CrossRef ] [ Google Scholar ]
- Shavrukov Y., Baho M., Lopato S., Langridge P. (2016). The TaDREB3 transgene transferred by conventional crossings to different genetic backgrounds of bread wheat improves drought tolerance . Plant Biotechnol. J . 14 , 313–322. 10.1111/pbi.12385 [ PubMed ] [ CrossRef ] [ Google Scholar ]
- Sigfridsson K. G. V., Bernát G., Mamedov F., Styring S. (2004). Molecular interference of Cd 2+ with photosystem II . Biochim. Biophys. Acta - Bioenerg . 1659 , 19–31. 10.1016/j.bbabio.2004.07.003 [ PubMed ] [ CrossRef ] [ Google Scholar ]
- Singh H. P., Mahajan P., Kaur S., Batish D. R., Kohli R. K. (2013). Chromium toxicity and tolerance in plants . Environ. Chem. Lett . 11 , 229–254. 10.1007/s10311-013-0407-5 [ CrossRef ] [ Google Scholar ]
- Smolikova G., Dolgikh E., Vikhnina M., Frolov A., Medvedev S. (2017). Genetic and hormonal regulation of chlorophyll degradation during maturation of seeds with green embryos . Int. J. Mol. Sci . 18 :1993. 10.3390/ijms18091993 [ PMC free article ] [ PubMed ] [ CrossRef ] [ Google Scholar ]
- Soares C., Branco-Neves S., de Sousa A., Azenha M., Cunha A., Pereira R., et al.. (2018). SiO2 nanomaterial as a tool to improve Hordeum vulgare L . tolerance to nano-NiO stress . Sci. Total Environ . 622–623 , 517–525. 10.1016/j.scitotenv.2017.12.002 [ PubMed ] [ CrossRef ] [ Google Scholar ]
- Soares C., Branco-Neves S., de Sousa A., Pereira R., Fidalgo F. (2016a). Ecotoxicological relevance of nano-NiO and acetaminophen to Hordeum vulgare L.: combining standardized procedures and physiological endpoints . Chemosphere 165 , 442–452. 10.1016/j.chemosphere.2016.09.053 [ PubMed ] [ CrossRef ] [ Google Scholar ]
- Soares C., Carvalho M. E. A., Azevedo R. A., Fidalgo F. (2019). Plants facing oxidative challenges—a little help from the antioxidant networks . Environ. Exp. Bot . 161 , 4–25. 10.1016/j.envexpbot.2018.12.009 [ CrossRef ] [ Google Scholar ]
- Soares C., de Sousa A., Pinto A., Azenha M., Teixeira J., Azevedo R. A., et al. (2016b). Effect of 24-epibrassinolide on ROS content, antioxidant system, lipid peroxidation and Ni uptake in Solanum nigrum L. under Ni stress . Environ. Exp. Bot . 122 :115–125. 10.1016/j.envexpbot.2015.09.010 [ CrossRef ] [ Google Scholar ]
- Solymosi K., Schoefs B. (2010). Etioplast and etio-chloroplast formation under natural conditions: the dark side of chlorophyll biosynthesis in angiosperms . Photosynth. Res . 105 , 143–166. 10.1007/s11120-010-9568-2 [ PubMed ] [ CrossRef ] [ Google Scholar ]
- Sonoike K. (2011). Photoinhibition of photosystem I . Physiol. Plant 142 , 56–64. 10.1111/j.1399-3054.2010.01437.x [ PubMed ] [ CrossRef ] [ Google Scholar ]
- Srivastava G., Kumar S., Dubey G., Mishra V., Prasad S. M. (2012). Nickel and ultraviolet-B stresses induce differential growth and photosynthetic responses in Pisum sativum L. seedlings . Biol. Trace Elem. Res . 149 , 86–96. 10.1007/s12011-012-9406-9 [ PubMed ] [ CrossRef ] [ Google Scholar ]
- Srivastava S., Fristensky B., Kav N. N. V. (2004). Constitutive expression of a PR10 protein enhances the germination of Brassica napus under saline conditions . Plant Cell Physiol . 45 , 1320–1324. 10.1093/pcp/pch137 [ PubMed ] [ CrossRef ] [ Google Scholar ]
- Staneloni R. J., Rodriguez-Batiller M. J., Casal J. J. (2008). Abscisic acid, high-light, and oxidative stress down-regulate a photosynthetic gene via a promoter motif not involved in phytochrome-mediated transcriptional regulation . Mol. Plant 1 , 75–83. 10.1093/mp/ssm007 [ PubMed ] [ CrossRef ] [ Google Scholar ]
- Streb P., Aubert S., Gout E., Bligny R. (2003). Reversibility of cold- and light-stress tolerance and accompanying changes of metabolite and antioxidant levels in the two high mountain plant species Soldanella alpina and Ranunculus glacialis . J. Exp. Bot . 54 , 405–418. 10.1093/jxb/erg048 [ PubMed ] [ CrossRef ] [ Google Scholar ]
- Streb P., Josse E. M., Gallouët E., Baptist F., Kuntz M., Cornic G. (2005). Evidence for alternative electron sinks to photosynthetic carbon assimilation in the high mountain plant species Ranunculus glacialis . Plant Cell Environ . 28 , 1123–1135. 10.1111/j.1365-3040.2005.01350.x [ CrossRef ] [ Google Scholar ]
- Sun J., Gu J., Zeng J., Han S., Song A., Chen F., et al. (2013). Changes in leaf morphology, antioxidant activity and photosynthesis capacity in two different drought-tolerant cultivars of chrysanthemum during and after water stress . Sci. Hortic . 161 , 249–258. 10.1016/j.scienta.2013.07.015 [ CrossRef ] [ Google Scholar ]
- Sun C., Liu L., Wang L., Li B., Jin C., Lin X. (2020). Melatonin: a master regulator of plant development and stress responses . J. Integr. Plant Biol . 1–20. 10.1111/jipb.12993 [ PubMed ] [ CrossRef ] [ Google Scholar ]
- Sundby C., Andersson B. (1985). Temperature-induced reversible migration along the thylakoid membrane of photosystem II regulates it association with LHC-II . FEBS Lett . 191 , 24–28. 10.1016/0014-5793(85)80986-8 [ CrossRef ] [ Google Scholar ]
- Taffouo V. D., Wamba O. F., Youmbi E., Nono G. V., Akoa A. (2010). Growth, yield, water status and ionic distribution response of three bambara groundnut ( Vigna subterranea (L.) verdc.) landraces grown under saline conditions . Int. J. Bot . 6 , 53–58. 10.3923/ijb.2010.53.58 [ CrossRef ] [ Google Scholar ]
- Taiz L., Zeiger E. (2010). Plant Physiology, 5th Edn . Sunderland, MA: Sinauer Associates, Inc. [ Google Scholar ]
- Takahashi S., Milward S. E., Milward D. Y. F., Chow W. S., Badger M. R. (2009). How does cyclic electron flow alleviate photoinhibition in arabidopsis? Plant Physiol . 149 , 1560–1567. 10.1104/pp.108.134122 [ PMC free article ] [ PubMed ] [ CrossRef ] [ Google Scholar ]
- Takahashi S., Murata N. (2008). How do environmental stresses accelerate photoinhibition? Trends Plant Sci . 13 , 178–182. 10.1016/j.tplants.2008.01.005 [ PubMed ] [ CrossRef ] [ Google Scholar ]
- Tamás M. J., Sharma S. K., Ibstedt S., Jacobson T., Christen P. (2014). Heavy metals and metalloids as a cause for protein misfolding and aggregation . Biomolecules 4 , 252–267. 10.3390/biom4010252 [ PMC free article ] [ PubMed ] [ CrossRef ] [ Google Scholar ]
- Tanveer M., Shahzad B., Sharma A., Khan E. A. (2019). 24-Epibrassinolide application in plants: an implication for improving drought stress tolerance in plants . Plant Physiol. Biochem . 135 , 295–303. 10.1016/j.plaphy.2018.12.013 [ PubMed ] [ CrossRef ] [ Google Scholar ]
- Tattini M., Velikova V., Vickers C., Brunetti C., Di Ferdinando M., Trivellini A., et al.. (2014). Isoprene production in transgenic tobacco alters isoprenoid, non-structural carbohydrate and phenylpropanoid metabolism, and protects photosynthesis from drought stress . Plant Cell Environ . 37 , 1950–1964. 10.1111/pce.12350 [ PubMed ] [ CrossRef ] [ Google Scholar ]
- Taub D. (2010). Effects of rising atmospheric concentrations of carbon dioxide on plants . Nat. Educ. Knowl . 3 :21. [ Google Scholar ]
- Tayefi-Nasrabadi H., Dehghan G., Daeihassani B., Movafegi A., Samadi A. (2011). Some biochemical properties of guaiacol peroxidases as modified by salt stress in leaves of salt-tolerant and salt-sensitive safflower ( Carthamus tinctorius L.cv.) cultivars . African J. Biotechnol . 10 , 751–763. 10.5897/AJB10.1465 [ CrossRef ] [ Google Scholar ]
- Teixeira R. N., Ligterink W., de França-Neto J. B., Hilhorst H. W. M., da Silva E. A. A. (2016). Gene expression profiling of the green seed problem in Soybean . BMC Plant Biol . 16 :37. 10.1186/s12870-016-0729-0 [ PMC free article ] [ PubMed ] [ CrossRef ] [ Google Scholar ]
- Tewari A. K., Tripathy B. C. (1998). Temperature-stress-induced impairment of chlorophyll biosynthetic reactions in cucumber and wheat . Plant Physiol . 117 , 851–858. 10.1104/pp.117.3.851 [ PMC free article ] [ PubMed ] [ CrossRef ] [ Google Scholar ]
- Tewari A. K., Tripathy B. C. (1999). Acclimation of chlorophyll biosynthetic reactions to temperature stress in cucumber ( Cucumis sativus L.) . Planta 208 , 431–437. 10.1007/s004250050579 [ CrossRef ] [ Google Scholar ]
- Thomashow M. F. (2010). Molecular basis of plant cold acclimation: insights gained from studying the CBF cold response pathway . Plant Physiol . 154 , 571–577. 10.1104/pp.110.161794 [ PMC free article ] [ PubMed ] [ CrossRef ] [ Google Scholar ]
- Tibiletti T., Auroy P., Peltier G., Caffarri S. (2016). Chlamydomonas reinhardtii PsbS protein is functional and accumulates rapidly and transiently under high light . Plant Physiol . 171 , 2717–2730. 10.1104/pp.16.00572 [ PMC free article ] [ PubMed ] [ CrossRef ] [ Google Scholar ]
- Tikkanen M., Gollan P. J., Mekala N. R., Isojärvi J., Aro E. M. (2014). Light-harvesting mutants show differential gene expression upon shift to high light as a consequence of photosynthetic redox and reactive oxygen species metabolism . Philos. Trans. R. Soc. B Biol. Sci . 369 :20130229. 10.1098/rstb.2013.0229 [ PMC free article ] [ PubMed ] [ CrossRef ] [ Google Scholar ]
- Tikkanen M., Grieco M., Nurmi M., Rantala M., Suorsa M., Aro E. M. (2012). Regulation of the photosynthetic apparatus under fluctuating growth light . Philos. Trans. R. Soc. B Biol. Sci . 367 , 3486–3493. 10.1098/rstb.2012.0067 [ PMC free article ] [ PubMed ] [ CrossRef ] [ Google Scholar ]
- Tóth S. Z., Nagy V., Puthur J. T., Kovács L., Garab G. (2011). The physiological role of ascorbate as photosystem II electron donor: protection against photoinactivation in heat-stressed leaves . Plant Physiol . 156 , 382–392. 10.1104/pp.110.171918 [ PMC free article ] [ PubMed ] [ CrossRef ] [ Google Scholar ]
- Turk H. (2019). Chitosan-induced enhanced expression and activation of alternative oxidase confer tolerance to salt stress in maize seedlings . Plant Physiol. Biochem . 141 , 415–422. 10.1016/j.plaphy.2019.06.025 [ PubMed ] [ CrossRef ] [ Google Scholar ]
- Tutej N., Tarique M., Tutej R. (2014). Rice SUV3 is a bidirectional helicase that binds both DNA and RNA . BMC Plant Biol . 14 :283 10.1186/s12870-014-0283-6 [ PMC free article ] [ PubMed ] [ CrossRef ] [ Google Scholar ]
- Upadhyaya C. P., Venkatesh J., Gururani M. A., Asnin L., Sharma K., Ajappala H., et al.. (2011). Transgenic potato overproducing l-ascorbic acid resisted an increase in methylglyoxal under salinity stress via maintaining higher reduced glutathione level and glyoxalase enzyme activity . Biotechnol. Lett . 33 , 2297–2307. 10.1007/s10529-011-0684-7 [ PubMed ] [ CrossRef ] [ Google Scholar ]
- Vassilev A., Nikolova A., Koleva L., Lidon F. (2011). Effects of excess Zn on growth and photosynthetic performance of young bean plants . J. Phytol . 3 , 58–62. [ Google Scholar ]
- Vassilev A., Schwitzguebel J. P., Thewys T., Van Der Lelie D., Vangronsveld J. (2004). The use of plants for remediation of metal-contaminated soils . Sci. World J . 4 , 9–34. 10.1100/tsw.2004.2 [ PMC free article ] [ PubMed ] [ CrossRef ] [ Google Scholar ]
- Veiga T. A. M., Silva S. C., Francisco A. C., Filho E. R., Vieira P. C., Fernandes J. B., et al.. (2007). Inhibition of photophosphorylation and electron transport chain in thylakoids by lasiodiplodin, a natural product from Botryosphaeria rhodina . J. Agricult. Food Chem . 55 , 4217–4221. 10.1021/jf070082b [ PubMed ] [ CrossRef ] [ Google Scholar ]
- Velikova V., Sharkey T. D., Loreto F. (2012). Stabilization of thylakoid membranes in isoprene-emitting plants reduces formation of reactive oxygen species . Plant Signal. Behav . 7 , 139–141. 10.4161/psb.7.1.18521 [ PMC free article ] [ PubMed ] [ CrossRef ] [ Google Scholar ]
- Vieira Santos C. L., Campos A., Azevedo H., Caldeira G. (2001). In situ and in vitro senescence induced by KCI stress: nutritional imbalance, lipid peroxidation and antioxidant metabolism . J. Exp. Bot . 52 , 351–360. 10.1093/jexbot/52.355.351 [ PubMed ] [ CrossRef ] [ Google Scholar ]
- Voigt C., Oster U., Bornke F., Jahns P., Dietz K. J., Leister D., et al. (2010). In-depth analysis of the distinctive effects ofnorflurazon implies that tetrapyrrole biosynthesis, organellar geneexpression and ABA cooperate in the GUN-type of plastid signalling . Physiol. Plant . 138 , 503–519. 10.1111/j.1399-3054.2009.01343.x [ PubMed ] [ CrossRef ] [ Google Scholar ]
- von Caemmerer S., Farquhar G. D. (1984). Effects of partial defoliation, changes of irradiance during growth, short-term water stress and growth at enhanced p(CO 2 ) on the photosynthetic capacity of leaves of Phaseolus vulgaris L . Planta 160 , 320–329. 10.1007/BF00393413 [ PubMed ] [ CrossRef ] [ Google Scholar ]
- Wan G., Najeeb U., Jilani G., Naeem M. S., Zhou W. (2011). Calcium invigorates the cadmium-stressed Brassica napus L. plants by strengthening their photosynthetic system . Environ. Sci. Pollut. Res . 18 , 1478–1486. 10.1007/s11356-011-0509-1 [ PubMed ] [ CrossRef ] [ Google Scholar ]
- Wang D., Li X. F., Zhou Z. J., Feng X. P., Yang W. J., Jiang D. A. (2010). Two Rubisco activase isoforms may play different roles in photosynthetic heat acclimation in the rice plant . Physiol. Plant 139 , 55–67. 10.1111/j.1399-3054.2009.01344.x [ PubMed ] [ CrossRef ] [ Google Scholar ]
- Wang L., Deng F., Ren W. J., Yang W. Y. (2013). Effects of shading on starch pasting characteristics of indica hybrid rice ( Oryza sativa L.) . PLoS ONE 8 :e68220. 10.1371/journal.pone.0068220 [ PMC free article ] [ PubMed ] [ CrossRef ] [ Google Scholar ]
- Wang L., Feng C., Zheng X., Guo Y., Zhou F., Shan D., et al.. (2017). Plant mitochondria synthesize melatonin and enhance the tolerance of plants to drought stress . J. Pineal Res . 63 , 1–11. 10.1111/jpi.12429 [ PubMed ] [ CrossRef ] [ Google Scholar ]
- Wang L., Liu J., Wang W., Sun Y. (2016). Exogenous melatonin improves growth and photosynthetic capacity of cucumber under salinity-induced stress . Photosynthetica 54 , 19–27. 10.1007/s11099-015-0140-3 [ CrossRef ] [ Google Scholar ]
- Wang L. J., Fan L., Loescher W., Duan W., Liu G. J., Cheng J. S., et al.. (2010). Salicylic acid alleviates decreases in photosynthesis under heat stress and accelerates recovery in grapevine leaves . BMC Plant Biol . 10 , 34–44. 10.1186/1471-2229-10-34 [ PMC free article ] [ PubMed ] [ CrossRef ] [ Google Scholar ]
- Wang Q., Liu Q., Wang X., Zuo Z., Oka Y., Lin C. (2018). New insights into the mechanisms of phytochrome–cryptochrome coaction . New Phytol . 217 , 547–551. 10.1111/nph.14886 [ PMC free article ] [ PubMed ] [ CrossRef ] [ Google Scholar ]
- Wang Y., Tong Y., Chu H., Chen X., Guo H., Yuan H., et al. (2017). Effects of different light qualities on seedling growth and chlorophyll fluorescence parameters of Dendrobium officinale . Biologia 72 , 735–744. 10.1515/biolog-2017-0081 [ CrossRef ] [ Google Scholar ]
- Waters M. T., Wang P., Korkaric M., Capper R. G., Saunders N. J., Langdale J. A. (2009). GLK transcription factors coordinate expression of the photosynthetic apparatus in Arabidopsis . Plant Cell 21 , 1109–1128. 10.1105/tpc.108.065250 [ PMC free article ] [ PubMed ] [ CrossRef ] [ Google Scholar ]
- Wei W., Li Q.-T., Chu Y.-N., Reiter R. J., Yu X.-M., Zhu D.-H., et al.. (2014). Melatonin enhances plant growth and abiotic stress tolerance in soybean plants . J. Exper. Bot . 66 , 695–707. 10.1093/jxb/eru392 [ PMC free article ] [ PubMed ] [ CrossRef ] [ Google Scholar ]
- Winicov I., Seemann J. R. (1990). Expression of genes for photosynthesis and the relationship to salt tolerance of alfalfa ( Medicago sativa ) cells . Plant Cell Physiol . 31 , 1155–1161. 10.1093/oxfordjournals.pcp.a078029 [ CrossRef ] [ Google Scholar ]
- Wu Q. S., Zou Y. N. (2009). Adaptive responses of birch-leaved pear ( Pyrus betulaefolia ) seedlings to salinity stress . Not. Bot. Horti Agrobot. Cluj Napoca 37 , 133–138. 10.15835/nbha3713109 [ CrossRef ] [ Google Scholar ]
- Xia X. J., Huang Y. Y., Wang L., Huang L. F., Yu Y. L., Zhou Y. H., et al. (2006). Pesticides-induced depression of photosynthesis was alleviated by 24-epibrassinolide pretreatment in Cucumis sativus L . Pestic. Biochem. Physiol . 86 , 42–48. 10.1016/j.pestbp.2006.01.005 [ CrossRef ] [ Google Scholar ]
- Xiao X., Yang F., Zhang S., Korpelainen H., Li C. (2009). Physiological and proteomic responses of two contrasting Populus cathayana populations to drought stress . Physiol. Plant . 136 , 150–168. 10.1111/j.1399-3054.2009.01222.x [ PubMed ] [ CrossRef ] [ Google Scholar ]
- Xu W., Cai S. Y., Zhang Y., Wang Y., Ahammed G. J., Xia X. J., et al.. (2016a). Melatonin enhances thermotolerance by promoting cellular protein protection in tomato plants . J. Pineal Res . 61 , 457–469. 10.1111/jpi.12359 [ PubMed ] [ CrossRef ] [ Google Scholar ]
- Xu W., Zhou Y., Chollet R. (2003). Identification and expression of a soybean nodule-enhanced PEP-carboxylase kinase gene (NE-PpcK) that shows striking up-/down-regulation in vivo . Plant J . 34 :441–452. 10.1046/j.1365-313X.2003.01740.x [ PubMed ] [ CrossRef ] [ Google Scholar ]
- Xu X. D., Sun Y., Sun B., Zhang J., Guo X. Q. (2010). Effects of exogenous melatonin on active oxygen metabolism of cucumber seedlings under high temperature stress . Chinese J. Appl. Ecol . 21 , 1295–300. [ PubMed ] [ Google Scholar ]
- Xu Y., Ibrahim I. M., Harvey P. J. (2016b). The influence of photoperiod and light intensity on the growth and photosynthesis of Dunaliella salina (chlorophyta) CCAP 19/30 . Plant Physiol. Biochem . 106 , 305–315. 10.1016/j.plaphy.2016.05.021 [ PMC free article ] [ PubMed ] [ CrossRef ] [ Google Scholar ]
- Xu Y. H., Liu R., Yan L., Liu Z. Q., Jiang S. C., Shen Y. Y., et al.. (2012). Light-harvesting chlorophyll a/b-binding proteins are required for stomatal response to abscisic acid in Arabidopsis . J. Exp. Bot . 63 , 1095–1106. 10.1093/jxb/err315 [ PMC free article ] [ PubMed ] [ CrossRef ] [ Google Scholar ]
- Xue G. P., McIntyre C. L., Glassop D., Shorter R. (2008). Use of expression analysis to dissect alterations in carbohydrate metabolism in wheat leaves during drought stress . Plant Mol. Biol . 67 , 197–214. 10.1007/s11103-008-9311-y [ PubMed ] [ CrossRef ] [ Google Scholar ]
- Yadav P., Kaur R., Kanwar M. K., Sharma A., Verma V., Sirhindi G., et al.. (2018). Castasterone confers copper stress tolerance by regulating antioxidant enzyme responses, antioxidants, and amino acid balance in B. juncea seedlings . Ecotoxicol. Environ. Saf . 147 , 725–734. 10.1016/j.ecoenv.2017.09.035 [ PubMed ] [ CrossRef ] [ Google Scholar ]
- Yamori W., Hikosaka K., Way D. A. (2014). Temperature response of photosynthesis in C3, C4, and CAM plants: temperature acclimation and temperature adaptation . Photosynth. Res . 119 , 101–117. 10.1007/s11120-013-9874-6 [ PubMed ] [ CrossRef ] [ Google Scholar ]
- Yanagisawa S. (2000). Dof1 and Dof2 transcription factors are associated with expression of multiple genes involved in carbon metabolism in maize . Plant J . 21 , 281–288. 10.1046/j.1365-313X.2000.00685.x [ PubMed ] [ CrossRef ] [ Google Scholar ]
- Yanagisawa S., Sheen J. (1998). Involvement of maize Dof zinc finger proteins in tissue-specific and light-regulated gene expression . Plant Cell . 10 , 75–89. 10.1105/tpc.10.1.75 [ PMC free article ] [ PubMed ] [ CrossRef ] [ Google Scholar ]
- Yang W. J., Du Y. T., Zhou Y., Bin C. J., Xu Z. S., Ma Y. Z., et al.. (2019). Overexpression of TaCOMT improves melatonin production and enhances drought tolerance in transgenic Arabidopsis . Int. J. Mol. Sci . 20 :652. 10.3390/ijms20030652 [ PMC free article ] [ PubMed ] [ CrossRef ] [ Google Scholar ]
- Yang X., Liang Z., Lu C. (2005). Genetic engineering of the biosynthesis of glycinebetaine enhances photosynthesis against high temperature stress in transgenic tobacco plants . Plant Physiol . 138 , 2299–2309. 10.1104/pp.105.063164 [ PMC free article ] [ PubMed ] [ CrossRef ] [ Google Scholar ]
- Yang X. L., Xu H., Li D., Gao X., Li T. L., Wang R. (2018). Effect of melatonin priming on photosynthetic capacity of tomato leaves under low-temperature stress . Photosynthetica 56 , 884–892. 10.1007/s11099-017-0748-6 [ CrossRef ] [ Google Scholar ]
- Ye J., Wang S., Deng X., Yin L., Xiong B., Wang X. (2016). Melatonin increased maize ( Zea mays L.) seedling drought tolerance by alleviating drought-induced photosynthetic inhibition and oxidative damage . Acta Physiol. Plant . 38 :48 10.1007/s11738-015-2045-y [ CrossRef ] [ Google Scholar ]
- Yoshioka M., Uchida S., Mori H., Komayama K., Ohira S., Morita N., et al. (2006). Quality control of photosystem II: cleavage of reaction center D1 protein in spinach thylakoids by FtsH protease under moderate heat stress . J. Biol. Chem . 281 , 21660–21669. 10.1074/jbc.M602896200 [ PubMed ] [ CrossRef ] [ Google Scholar ]
- Yu X. Z., Lin Y. J., Zhang Q. (2019). Metallothioneins enhance chromium detoxification through scavenging ROS and stimulating metal chelation in Oryza sativa . Chemosphere 220 , 300–313. 10.1016/j.chemosphere.2018.12.119 [ PubMed ] [ CrossRef ] [ Google Scholar ]
- Yuan L., Xu D. Q. (2001). Stimulation effect of gibberellic acid short-term treatment on leaf photosynthesis related to the increase in Rubisco content in broad bean and soybean . Photosynth. Res . 68 , 39–47. 10.1023/A:1011894912421 [ PubMed ] [ CrossRef ] [ Google Scholar ]
- Zhang H., Xu Z., Guo K., Huo Y., He G., Sun H., et al.. (2020). Toxic effects of heavy metal Cd and Zn on chlorophyll, carotenoid metabolism and photosynthetic function in tobacco leaves revealed by physiological and proteomics analysis . Ecotoxicol. Environ. Saf . 202 :110856. 10.1016/j.ecoenv.2020.110856 [ PubMed ] [ CrossRef ] [ Google Scholar ]
- Zhang H., Zhong H., Wang J., Sui X., Xu N. (2016). Adaptive changes in chlorophyll content and photosynthetic features to low light in Physocarpus amurensis Maxim and Physocarpus opulifolius “Diabolo.” PeerJ 4 :e2125. 10.7717/peerj.2125 [ PMC free article ] [ PubMed ] [ CrossRef ] [ Google Scholar ]
- Zhang J., Shi Y., Zhang X., Du H., Xu B., Huang B. (2017). Melatonin suppression of heat-induced leaf senescence involves changes in abscisic acid and cytokinin biosynthesis and signaling pathways in perennial ryegrass ( Lolium perenne L.) . Environ. Exp. Bot . 138 , 36–45. 10.1016/j.envexpbot.2017.02.012 [ CrossRef ] [ Google Scholar ]
- Zhang L., Guo X., Zhang Z., Wang A., Zhu J. (2021). Cold-regulated gene LeCOR413PM2 confers cold stress tolerance in tomato plants . Gene 764 :145097 10.1016/j.gene.2020.145097 [ PubMed ] [ CrossRef ] [ Google Scholar ]
- Zhang L. T., Zhang Z. S., Gao H. Y., Xue Z. C., Yang C., Meng X. L., et al.. (2011). Mitochondrial alternative oxidase pathway protects plants against photoinhibition by alleviating inhibition of the repair of photodamaged PSII through preventing formation of reactive oxygen species in Rumex K-1 leaves . Physiol. Plant . 143 , 396–407. 10.1111/j.1399-3054.2011.01514.x [ PubMed ] [ CrossRef ] [ Google Scholar ]
- Zhang M., Pan J., Kong X., Zhou Y., Liu Y., Sun L., et al.. (2012). ZmMKK3, a novel maize group B mitogen-activated protein kinase kinase gene, mediates osmotic stress and ABA signal responses . J. Plant Physiol . 169 , 1501–1510. 10.1016/j.jplph.2012.06.008 [ PubMed ] [ CrossRef ] [ Google Scholar ]
- Zhang Q. (2007). Strategies for developing green super rice . Proc. Natl. Acad. Sci. U.S.A . 104 :16402. 10.1073/pnas.0708013104 [ PMC free article ] [ PubMed ] [ CrossRef ] [ Google Scholar ]
- Zhang S., Scheller H. V. (2004). Photoinhibition of photosystem I at chilling temperature and subsequent recovery in Arabidopsis thaliana . Plant Cell Physiol . 45 , 1595–1602. 10.1093/pcp/pch180 [ PubMed ] [ CrossRef ] [ Google Scholar ]
- Zhang X., Wollenweber B., Jiang D., Liu F., Zhao J. (2008). Water deficits and heat shock effects on photosynthesis of a transgenic Arabidopsis thaliana constitutively expressing ABP9, a bZIP transcription factor . J. Exp. Bot . 59 , 839–848. 10.1093/jxb/erm364 [ PubMed ] [ CrossRef ] [ Google Scholar ]
- Zhang X., Zhang X., Gao B., Li Z., Xia H., Li H., et al.. (2014). Effect of cadmium on growth, photosynthesis, mineral nutrition and metal accumulation of anenergy crop, king grass ( Pennisetum americanum × P. purpureum ) . Biomass Bioenergy . 67 , 179–187. 10.1016/j.biombioe.2014.04.030 [ PubMed ] [ CrossRef ] [ Google Scholar ]
- Zhao G., Yu X., Lou W., Wei S., Wang R., Wan Q., et al. (2019). Transgenic Arabidopsis overexpressing MsSNAT enhances salt tolerance via the increase in autophagy, and the reestablishment of redox and ion homeostasis . Environ. Exp. Bot . 164 , 20–28. 10.1016/j.envexpbot.2019.04.017 [ CrossRef ] [ Google Scholar ]
- Zhao H.-Z., Zhao X.-Z., Ma P.-F., Wang Y.-X., Hu W.-W., Li L.-H., et al. (2011). Effects of salicylic acid on protein kinase activity and chloroplast D1 protein degradation in wheat leaves subjected to heat and high light stress . Acta Ecol. Sin . 31 , 259–263. 10.1016/j.chnaes.2011.06.006 [ CrossRef ] [ Google Scholar ]
- Zheng X., Zhou J., Tan D. X., Wang N., Wang L., Shan D., et al.. (2017). Melatonin improves waterlogging tolerance of Malus baccata (Linn.) borkh. seedlings by maintaining aerobic respiration, photosynthesis and ROS migration . Front. Plant Sci . 8 :483. 10.3389/fpls.2017.00483 [ PMC free article ] [ PubMed ] [ CrossRef ] [ Google Scholar ]
- Zhou B., Peng D., Lin J., Huang X., Peng W., He R., et al. (2011). Heterologous expression of a gibberellin 2-oxidase gene from Arabidopsis thaliana enhanced the photosynthesis capacity in Brassica napus L . J. Plant Biol . 54 , 23–32. 10.1007/s12374-010-9139-2 [ CrossRef ] [ Google Scholar ]
- Zhou X., Zhao H., Cao K., Hu L., Du T., Balušk, et al.. (2016). Beneficial roles of melatonin on redox regulation of photosynthetic electron transport and synthesis of D1 protein in tomato seedlings under salt stress . Front. Plant Sci . 7 :1823. 10.3389/fpls.2016.01823 [ PMC free article ] [ PubMed ] [ CrossRef ] [ Google Scholar ]
- Zhu S. Q., Chen M. W., Ji B. H., Jiao D. M., Liang J. S. (2011). Roles of xanthophylls and exogenous ABA in protection against NaCl-induced photodamage in rice ( Oryza sativa L.) and cabbage ( Brassica campestris ) . J. Exp. Bot . 62 , 4617–4625. 10.1093/jxb/err170 [ PubMed ] [ CrossRef ] [ Google Scholar ]
- Ziaf K., Amjad M., Pervez M. A., Iqbal Q., Rajwana I. A., Ayyub M. (2009). Evaluation of different growth and physiological traits as indices of salt tolerance in hot pepper ( Capsicum annuum L.) . Pakistan J. Bot . 41 , 1797–1809. [ Google Scholar ]
- Zong H., Liu S., Xing R., Chen X., Li P. (2017). Protective effect of chitosan on photosynthesis and antioxidative defense system in edible rape ( Brassica rapa L.) in the presence of cadmium . Ecotoxicol. Environ. Saf . 138 , 271–278. 10.1016/j.ecoenv.2017.01.009 [ PubMed ] [ CrossRef ] [ Google Scholar ]
- Zong X. J., Li D. P., Gu L. K., Li D. Q., Liu L. X., Hu X. L. (2009). Abscisic acid and hydrogen peroxide induce a novel maize group C MAP kinase gene, ZmMPK7, which is responsible for the removal of reactive oxygen species . Planta 229 , 485–95. 10.1007/s00425-008-0848-4 [ PubMed ] [ CrossRef ] [ Google Scholar ]
- Zörb C., Herbst R., Forreiter C., Schubert S. (2009). Short-term effects of salt exposure on the maize chloroplast protein pattern . Proteomics 9 , 4209–4220. 10.1002/pmic.200800791 [ PubMed ] [ CrossRef ] [ Google Scholar ]
- Zuo B., Zheng X., He P., Wang L., Lei Q., Feng C., et al.. (2014). Overexpression of MzASMT improves melatonin production and enhances drought tolerance in transgenic Arabidopsis thaliana plants . J. Pineal Res . 57 , 408–417. 10.1111/jpi.12180 [ PubMed ] [ CrossRef ] [ Google Scholar ]
- Zuo Z., Sun L., Wang T., Miao P., Zhu X., Liu S., et al.. (2017). Melatonin improves the photosynthetic carbon assimilation and antioxidant capacity in wheat exposed to nano-zno stress . Molecules 22 :1727. 10.3390/molecules22101727 [ PMC free article ] [ PubMed ] [ CrossRef ] [ Google Scholar ]

COMMENTS
Abstract. Plants cannot move, so they must endure abiotic stresses such as drought, salinity and extreme temperatures. These stressors greatly limit the distribution of plants, alter their growth ...
1. Introduction. For immobile plants, abiotic environmental factors are often the main detrimental factors affecting their growth and development [].Abiotic stress refers to the adverse effect of any abiotic factor on a plant in a given environment, resulting in a range of responses, from changes affecting biological processes such as gene expression and cell metabolism to growth and ...
Biotic and abiotic environmental stressors are major factors that can limit plant growth and productivity, leading to significant losses in yield and biodiversity ( Nawaz et al., 2021; Rillig et al., 2019; Zohaib et al., 2018 ). Biotic stress is caused by insect pests, diseases, and competition with other plants, while abiotic stress refers to ...
The natural environment for plants is composed of a complex set of abiotic stresses and biotic stresses. Plant responses to these stresses are equally complex. Systems biology approaches facilitate a multi-targeted approach by allowing one to identify regulatory hubs in complex networks. Systems biology takes the molecular parts (transcripts, proteins and metabolites) of an organism and ...
From a meta-analysis of drought-stress related papers from the last 15 years, ... Despite the vast amount of research collected on abiotic stress in the last decade, there are still significant gaps in our knowledge. We still do not understand completely how plants perceive stress. We don't know all of the receptors and their sites of action ...
With the increasing impact of climate change and global warming, not only abiotic stress factors have gained prominence, but their influence on plant-biotic interaction has also increased. Plants respond differently to abiotic factors compared to pests and pathogens, which thrive under intense climatic conditions, leading to higher disease susceptibility and potential epidemic outbreaks ...
Here, we focus on ROS signalling, calcium signal-ling and protein phosphorylation, which are three major features of signal transduction in plants in response to many abiotic stresses. Although ...
Silicon amends biotic and abiotic stress situations in plants by regulating the physiological, biochemical, and molecular responses. Therefore, another review on the "Multidimensional role of silicon to activate resilient plant growth and to mitigate abiotic stress" by Mir et al. was included in this Research Topic. All of these reviews ...
Abiotic stress conditions such as drought, high temperatures, salinity, ... In this context, integrated omics research has been widely used to understand the plant's biological networking and molecular mechanisms against various abiotic stresses. Despite tremendous progress in genomics, there is a need to study other omics levels, including ...
Abstract. Plants cannot move, so they must endure abiotic stresses such as drought, salinity and extreme temperatures. These stressors greatly limit the distribution of plants, alter their growth and development, and reduce crop productivity. Recent progress in our understanding of the molecular mechanisms underlying the responses of plants to ...
long-observed phenomenon that abiotic stressors induce different signals and effects at the level. of gene expression, but genes whose regulation is similar under most stressors can still be ...
Abiotic stress is defined as the negative impact of non-living factors on the living organisms in a specific environment. The non-living variable... | Explore the latest full-text research PDFs ...
The major abiotic stresses (drought, high salinity, cold, and heat) negatively influence the survival, biomass production and yields of staple food crops up to 70% (Vorasoot et al. 2003; Kaur et al. 2008; Thakur et al. 2010) hence, threaten the food security worldwide.Dehydration stress imparted by drought, salinity and temperature severity is the most prevalent abiotic stress that limits ...
Figure 1. The general defense systems and the underlying regulatory network in botanic responses to abiotic stresses. Different abiotic stresses, such as cold, heat, drought, flood, and salt can provoke common cellular disorder and secondary stresses, including membrane injury, reactive species (RS) damage, protein denaturation, and osmotic stress, which are also interconnected with each other.
The study of abiotic stress in crop plants covers a gamut of approaches, ranging from environmental research through physiological investigations and molecular and genetic aspects of plant responses to stresses. ... The paper by Bal et al. analyses the impact of large-scale weather patterns across different environments on the yield of one rice ...
This Special Issue, under the title "Abiotic Stress Signaling and Responses in Plants", is a miscellany of twelve papers (both original research and reviews) related to plant signaling under various abiotic stress factors. Go to: 2. Salt Stress. In the study of Wang et al. [ 1 ], the authors delved into the effects of salt stress on plant ...
Plant stress factors are classified into two categories: abiotic and biotic (Umar et al., 2021). Abiotic factors are environmental factors that affect plant growth such as heat stress, drought ...
5 Advanced techniques in abiotic stress research - Shen et al. discovered that precisely introducing a regulatory element into a target gene's 5'UTR might effectively boost the protein abundance of that gene in rice. The research offered a fresh method to control protein translation for crop breeding by demonstrating the viability of such ...
"Abiotic stresses -; as opposed to biotic stresses like pests or disease -; such as drought, salinity and cold negatively affect plant growth and crop productivity.
Schematic representation of the photosynthesis performance under abiotic stresses (heat, drought, and salinity). Drought and heat stress down-regulate enzymatic activity and electron transport chain (ETC) and cause membrane rupture, low CO 2 solubility, leaf senescence, and reactive oxygen species (ROS) production. On the other hand, salinity causes ion toxicity, membrane disruption, reduced ...
Plant str ess can be divide d into tw o primary categories namely a biotic. stress and bio tic stress. Abiotic str ess imposed on plants by en vironmen t may be. either ph ysical or chemical ...
Zinc (Zn) is an essential micronutrient for plant health, yet its availability in soils grapples with limitations imposed by diverse factors. Nanotechnology offers a promising approach to enhance Zn delivery and uptake by plants, as well as to improve their tolerance to abiotic stress. However, the current literature lacks a comprehensive overview of the research trends and progress on Zn ...
It is our honor to present the highly accessed papers published by Horticulturae (ISSN: 2311-7524) in 2023 in the Section "Biotic and Abiotic Stress". We would like to acknowledge the individuals and teams whose work inspires fellow researchers and influences the field of horticulture science.
Chapter 1: Cell signalling in plants during abiotic and biotic stress, Chapter. 2: Salinity stress induced metabolic changes and its management, Chapter 3: High temperature stress: responses ...