- Scoping Review
- Open access
- Published: 14 November 2021

Effectiveness and safety of SARS-CoV-2 vaccine in real-world studies: a systematic review and meta-analysis
- Qiao Liu 1 na1 ,
- Chenyuan Qin 1 , 2 na1 ,
- Min Liu 1 &
- Jue Liu ORCID: orcid.org/0000-0002-1938-9365 1 , 2
Infectious Diseases of Poverty volume 10 , Article number: 132 ( 2021 ) Cite this article
56k Accesses
204 Citations
373 Altmetric
Metrics details
To date, coronavirus disease 2019 (COVID-19) becomes increasingly fierce due to the emergence of variants. Rapid herd immunity through vaccination is needed to block the mutation and prevent the emergence of variants that can completely escape the immune surveillance. We aimed to systematically evaluate the effectiveness and safety of COVID-19 vaccines in the real world and to establish a reliable evidence-based basis for the actual protective effect of the COVID-19 vaccines, especially in the ensuing waves of infections dominated by variants.
We searched PubMed, Embase and Web of Science from inception to July 22, 2021. Observational studies that examined the effectiveness and safety of SARS-CoV-2 vaccines among people vaccinated were included. Random-effects or fixed-effects models were used to estimate the pooled vaccine effectiveness (VE) and incidence rate of adverse events after vaccination, and their 95% confidence intervals ( CI ).
A total of 58 studies (32 studies for vaccine effectiveness and 26 studies for vaccine safety) were included. A single dose of vaccines was 41% (95% CI : 28–54%) effective at preventing SARS-CoV-2 infections, 52% (31–73%) for symptomatic COVID-19, 66% (50–81%) for hospitalization, 45% (42–49%) for Intensive Care Unit (ICU) admissions, and 53% (15–91%) for COVID-19-related death; and two doses were 85% (81–89%) effective at preventing SARS-CoV-2 infections, 97% (97–98%) for symptomatic COVID-19, 93% (89–96%) for hospitalization, 96% (93–98%) for ICU admissions, and 95% (92–98%) effective for COVID-19-related death, respectively. The pooled VE was 85% (80–91%) for the prevention of Alpha variant of SARS-CoV-2 infections, 75% (71–79%) for the Beta variant, 54% (35–74%) for the Gamma variant, and 74% (62–85%) for the Delta variant. The overall pooled incidence rate was 1.5% (1.4–1.6%) for adverse events, 0.4 (0.2–0.5) per 10 000 for severe adverse events, and 0.1 (0.1–0.2) per 10 000 for death after vaccination.
Conclusions
SARS-CoV-2 vaccines have reassuring safety and could effectively reduce the death, severe cases, symptomatic cases, and infections resulting from SARS-CoV-2 across the world. In the context of global pandemic and the continuous emergence of SARS-CoV-2 variants, accelerating vaccination and improving vaccination coverage is still the most important and urgent matter, and it is also the final means to end the pandemic.
Graphical Abstract
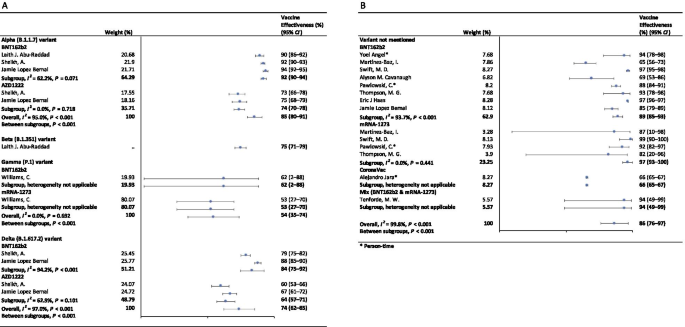
Since its outbreak, coronavirus disease 2019 (COVID-19) has spread rapidly, with a sharp rise in the accumulative number of infections worldwide. As of August 8, 2021, COVID-19 has already killed more than 4.2 million people and more than 203 million people were infected [ 1 ]. Given its alarming-spreading speed and the high cost of completely relying on non-pharmaceutical measures, we urgently need safe and effective vaccines to cover susceptible populations and restore people’s lives into the original [ 2 ].
According to global statistics, as of August 2, 2021, there are 326 candidate vaccines, 103 of which are in clinical trials, and 19 vaccines have been put into normal use, including 8 inactivated vaccines and 5 protein subunit vaccines, 2 RNA vaccines, as well as 4 non-replicating viral vector vaccines [ 3 ]. Our World in Data simultaneously reported that 27.3% of the world population has received at least one dose of a COVID-19 vaccine, and 13.8% is fully vaccinated [ 4 ].
To date, COVID-19 become increasingly fierce due to the emergence of variants [ 5 , 6 , 7 ]. Rapid herd immunity through vaccination is needed to block the mutation and prevent the emergence of variants that can completely escape the immune surveillance [ 6 , 8 ]. Several reviews systematically evaluated the effectiveness and/or safety of the three mainstream vaccines on the market (inactivated virus vaccines, RNA vaccines and viral vector vaccines) based on random clinical trials (RCT) yet [ 9 , 10 , 11 , 12 , 13 ].
In general, RNA vaccines are the most effective, followed by viral vector vaccines and inactivated virus vaccines [ 10 , 11 , 12 , 13 ]. The current safety of COVID-19 vaccines is acceptable for mass vaccination, but long-term monitoring of vaccine safety is needed, especially in older people with underlying conditions [ 9 , 10 , 11 , 12 , 13 ]. Inactivated vaccines had the lowest incidence of adverse events and the safety comparisons between mRNA vaccines and viral vectors were controversial [ 9 , 10 ].
RCTs usually conduct under a very demanding research circumstance, and tend to be highly consistent and limited in terms of population characteristics and experimental conditions. Actually, real-world studies differ significantly from RCTs in terms of study conditions and mass vaccination in real world requires taking into account factors, which are far more complex, such as widely heterogeneous populations, vaccine supply, willingness, medical accessibility, etc. Therefore, the real safety and effectiveness of vaccines turn out to be a major concern of international community. The results of a mass vaccination of CoronaVac in Chile demonstrated a protective effectiveness of 65.9% against the onset of COVID-19 after complete vaccination procedures [ 14 ], while the outcomes of phase 3 trials in Brazil and Turkey were 50.7% and 91.3%, reported on Sinovac’s website [ 14 ]. As for the Delta variant, the British claimed 88% protection after two doses of BNT162b2, compared with 67% for AZD1222 [ 15 ]. What is surprising is that the protection of BNT162b2 against infection in Israel is only 39% [ 16 ]. Several studies reported the effectiveness and safety of the COVID-19 vaccine in the real world recently, but the results remain controversial [ 17 , 18 , 19 , 20 ]. A comprehensive meta-analysis based upon the real-world studies is still in an urgent demand, especially for evaluating the effect of vaccines on variation strains. In the present study, we aimed to systematically evaluate the effectiveness and safety of the COVID-19 vaccine in the real world and to establish a reliable evidence-based basis for the actual protective effect of the COVID-19 vaccines, especially in the ensuing waves of infections dominated by variants.
Search strategy and selection criteria
Our methods were described in detail in our published protocol [PROSPERO (Prospective register of systematic reviews) registration, CRD42021267110]. We searched eligible studies published by 22 July 2021, from three databases including PubMed, Embase and Web of Science by the following search terms: (effectiveness OR safety) AND (COVID-19 OR coronavirus OR SARS-CoV-2) AND (vaccine OR vaccination). We used EndNoteX9.0 (Thomson ResearchSoft, Stanford, USA) to manage records, screen and exclude duplicates. This study was strictly performed according to the Preferred Reporting Items for Systematic Reviews and Meta-Analyses (PRISMA).
We included observational studies that examined the effectiveness and safety of severe acute respiratory syndrome coronavirus 2 (SARS-CoV-2) vaccines among people vaccinated with SARS-CoV-2 vaccines. The following studies were excluded: (1) irrelevant to the subject of the meta-analysis, such as studies that did not use SARS-CoV-2 vaccination as the exposure; (2) insufficient data to calculate the rate for the prevention of COVID-19, the prevention of hospitalization, the prevention of admission to the ICU, the prevention of COVID-19-related death, or adverse events after vaccination; (3) duplicate studies or overlapping participants; (4) RCT studies, reviews, editorials, conference papers, case reports or animal experiments; and (5) studies that did not clarify the identification of COVID-19.
Studies were identified by two investigators (LQ and QCY) independently following the criteria above, while discrepancies reconciled by a third investigator (LJ).
Data extraction and quality assessment
The primary outcome was the effectiveness of SARS-CoV-2 vaccines. The following data were extracted independently by two investigators (LQ and QCY) from the selected studies: (1) basic information of the studies, including first author, publication year and study design; (2) characteristics of the study population, including sample sizes, age groups, setting or locations; (3) kinds of the SARS-CoV-2 vaccines; (4) outcomes for the effectiveness of SARS-CoV-2 vaccines: the number of laboratory-confirmed COVID-19, hospitalization for COVID-19, admission to the ICU for COVID-19, and COVID-19-related death; and (5) outcomes for the safety of SARS-CoV-2 vaccines: the number of adverse events after vaccination.
We evaluated the risk of bias using the Newcastle–Ottawa quality assessment scale for cohort studies and case–control studies [ 21 ]. and assess the methodological quality using the checklist recommended by Agency for Healthcare Research and Quality (AHRQ) [ 22 ]. Cohort studies and case–control studies were classified as having low (≥ 7 stars), moderate (5–6 stars), and high risk of bias (≤ 4 stars) with an overall quality score of 9 stars. For cross-sectional studies, we assigned each item of the AHRQ checklist a score of 1 (answered “yes”) or 0 (answered “no” or “unclear”), and summarized scores across items to generate an overall quality score that ranged from 0 to 11. Low, moderate, and high risk of bias were identified as having a score of 8–11, 4–7 and 0–3, respectively.
Two investigators (LQ and QCY) independently assessed study quality, with disagreements resolved by a third investigator (LJ).
Data synthesis and statistical analysis
We performed a meta-analysis to pool data from included studies and assess the effectiveness and safety of SARS-CoV-2 vaccines by clinical outcomes (rates of the prevention of COVID-19, the prevention of hospitalization, the prevention of admission to the ICU, the prevention of COVID-19-related death, and adverse events after vaccination). Random-effects or fixed-effects models were used to pool the rates and adjusted estimates across studies separately, based on the heterogeneity between estimates ( I 2 ). Fixed-effects models were used if I 2 ≤ 50%, which represented low to moderate heterogeneity and random-effects models were used if I 2 > 50%, representing substantial heterogeneity.
We conducted subgroup analyses to investigate the possible sources of heterogeneity by using vaccine kinds, vaccination status, sample size, and study population as grouping variables. We used the Q test to conduct subgroup comparisons and variables were considered significant between subgroups if the subgroup difference P value was less than 0.05. Publication bias was assessed by funnel plot and Egger’s regression test. We analyzed data using Stata version 16.0 (StataCorp, Texas, USA).
A total of 4844 records were searched from the three databases. 2484 duplicates were excluded. After reading titles and abstracts, we excluded 2264 reviews, RCT studies, duplicates and other studies meeting our exclude criteria. Among the 96 studies under full-text review, 41 studies were excluded (Fig. 1 ). Ultimately, with three grey literatures included, this final meta-analysis comprised 58 eligible studies, including 32 studies [ 14 , 15 , 17 , 18 , 19 , 20 , 23 , 24 , 25 , 26 , 27 , 28 , 29 , 30 , 31 , 32 , 33 , 34 , 35 , 36 , 37 , 38 , 39 , 40 , 41 , 42 , 43 , 44 , 45 , 46 , 47 , 48 ] for vaccine effectiveness and 26 studies [ 49 , 50 , 51 , 52 , 53 , 54 , 55 , 56 , 57 , 58 , 59 , 60 , 61 , 62 , 63 , 64 , 65 , 66 , 67 , 68 , 69 , 70 , 71 , 72 , 73 , 74 ] for vaccine safety. Characteristics of included studies are showed in Additional file 1 : Table S1, Additional file 2 : Table S2. The risk of bias of all studies we included was moderate or low.
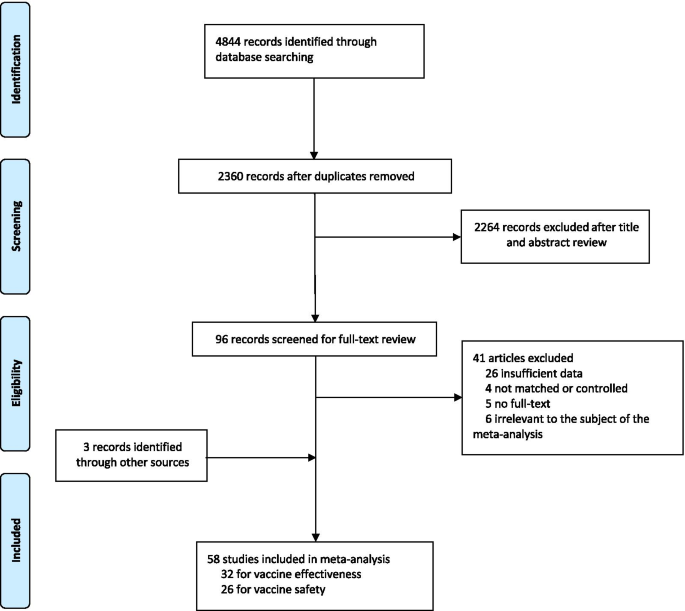
Flowchart of the study selection
Vaccine effectiveness for different clinical outcomes of COVID-19
We separately reported the vaccine effectiveness (VE) by the first and second dose of vaccines, and conducted subgroup analysis by the days after the first or second dose (< 7 days, ≥ 7 days, ≥ 14 days, and ≥ 21 days; studies with no specific days were classified as 1 dose, 2 dose or ≥ 1 dose).
For the first dose of SARS-CoV-2 vaccines, the pooled VE was 41% (95% CI : 28–54%) for the prevention of SARS-CoV-2 infection, 52% (95% CI : 31–73%) for the prevention of symptomatic COVID-19, 66% (95% CI : 50–81%) for the prevention of hospital admissions, 45% (95% CI : 42–49%) for the prevention of ICU admissions, and 53% (95% CI : 15–91%) for the prevention of COVID-19-related death (Table 1 ). The subgroup, ≥ 21 days after the first dose, was found to have the highest VE in each clinical outcome of COVID-19, regardless of ≥ 1 dose group (Table 1 ).
For the second dose of SARS-CoV-2 vaccines, the pooled VE was 85% (95% CI : 81–89%) for the prevention of SARS-CoV-2 infection, 97% (95% CI : 97–98%) for the prevention of symptomatic COVID-19, 93% (95% CI: 89–96%) for the prevention of hospital admissions, 96% (95% CI : 93–98%) for the prevention of ICU admissions, and 95% (95% CI : 92–98%) for the prevention of COVID-19-related death (Table 1 ). VE was 94% (95% CI : 78–98%) in ≥ 21 days after the second dose for the prevention of SARS-CoV-2 infection, higher than other subgroups, regardless of 2 dose group (Table 1 ). For the prevention of symptomatic COVID-19, VE was also relatively higher in 21 days after the second dose (99%, 95% CI : 94–100%). Subgroups showed no statistically significant differences in the prevention of hospital admissions, ICU admissions and COVID-19-related death (subgroup difference P values were 0.991, 0.414, and 0.851, respectively).
Vaccine effectiveness for different variants of SARS-CoV-2 in fully vaccinated people
In the fully vaccinated groups (over 14 days after the second dose), the pooled VE was 85% (95% CI: 80–91%) for the prevention of Alpha variant of SARS-CoV-2 infection, 54% (95% CI : 35–74%) for the Gamma variant, and 74% (95% CI : 62–85%) for the Delta variant. There was only one study [ 23 ] focused on the Beta variant, which showed the VE was 75% (95% CI : 71–79%) for the prevention of the Beta variant of SARS-CoV-2 infection. BNT162b2 vaccine had the highest VE in each variant group; 92% (95% CI : 90–94%) for the Alpha variant, 62% (95% CI : 2–88%) for the Gamma variant, and 84% (95% CI : 75–92%) for the Delta variant (Fig. 2 ).
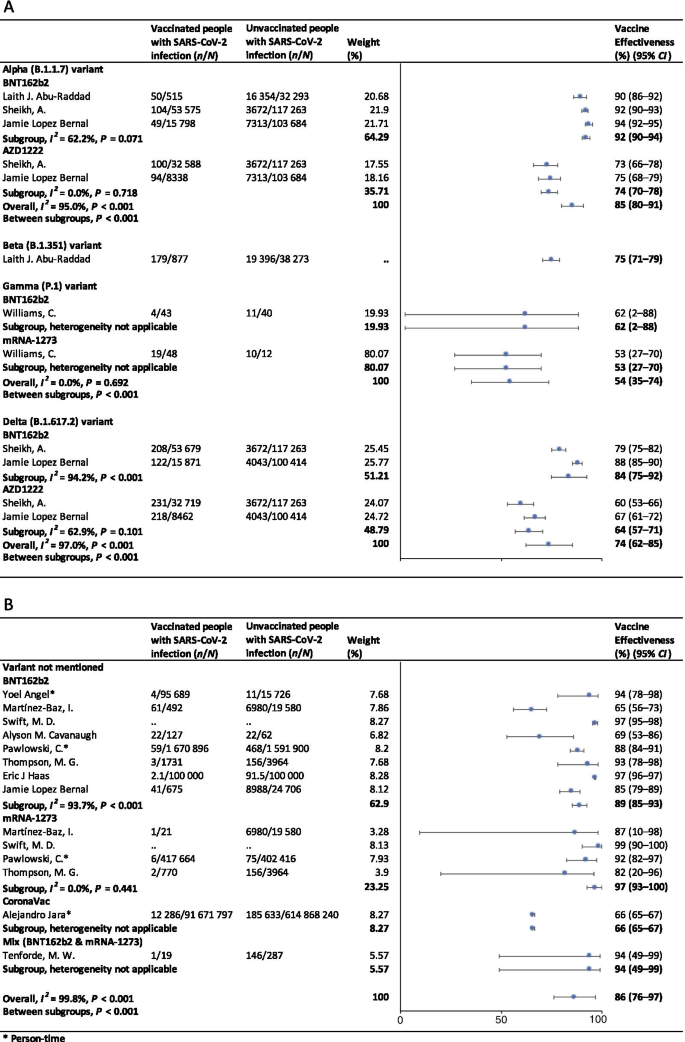
Forest plots for the vaccine effectiveness of SARS-CoV-2 vaccines in fully vaccinated populations. A Vaccine effectiveness against SARS-CoV-2 variants; B Vaccine effectiveness against SARS-CoV-2 with variants not mentioned. SARS-CoV-2 severe acute respiratory syndrome coronavirus 2, COVID-19 coronavirus disease 2019, CI confidence interval
For studies which had not mentioned the variant of SARS-CoV-2, the pooled VE was 86% (95% CI: 76–97%) for the prevention of SARS-CoV-2 infection in fully vaccinated people. mRNA-1273 vaccine had the highest pooled VE (97%, 95% CI: 93–100%, Fig. 2 ).
Safety of SARS-CoV-2 vaccines
As Table 2 showed, the incidence rate of adverse events varied widely among different studies. We conducted subgroup analysis by study population (general population, patients and healthcare workers), vaccine type (BNT162b2, mRNA-1273, CoronaVac, and et al.), and population size (< 1000, 1000–10 000, 10 000–100 000, and > 100 000). The overall pooled incidence rate was 1.5% (95% CI : 1.4–1.6%) for adverse events, 0.4 (95% CI : 0.2–0.5) per 10 000 for severe adverse events, and 0.1 (95% CI : 0.1–0.2) per 10 000 for death after vaccination. Incidence rate of adverse events was higher in healthcare workers (53.2%, 95% CI : 28.4–77.9%), AZD1222 vaccine group (79.6%, 95% CI : 60.8–98.3%), and < 1000 population size group (57.6%, 95% CI : 47.9–67.4%). Incidence rate of sever adverse events was higher in healthcare workers (127.2, 95% CI : 62.7–191.8, per 10 000), Gam-COVID-Vac vaccine group (175.7, 95% CI : 77.2–274.2, per 10 000), and 1000–10 000 population size group (336.6, 95% CI : 41.4–631.8, per 10 000). Incidence rate of death after vaccination was higher in patients (7.6, 95% CI : 0.0–32.2, per 10 000), BNT162b2 vaccine group (29.8, 95% CI : 0.0–71.2, per 10 000), and < 1000 population size group (29.8, 95% CI : 0.0–71.2, per 10 000). Subgroups of general population, vaccine type not mentioned, and > 100 000 population size had the lowest incidence rate of adverse events, severe adverse events, and death after vaccination.
Sensitivity analysis and publication bias
In the sensitivity analyses, VE for SARS-CoV-2 infections, symptomatic COVID-19 and COVID-19-related death got relatively lower when omitting over a single dose group of Maria et al.’s work [ 33 ]; when omitting ≥ 14 days after the first dose group and ≥ 14 days after the second dose group of Alejandro et al.’s work [ 14 ], VE for SARS-CoV-2 infections, hospitalization, ICU admission and COVID-19-related death got relatively higher; and VE for all clinical status of COVID-19 became lower when omitting ≥ 14 days after the second dose group of Eric et al.’s work [ 34 ]. Incidence rate of adverse events and severe adverse events got relatively higher when omitting China CDC’s data [ 74 ]. P values of Egger’s regression test for all the meta-analysis were more than 0.05, indicating that there might not be publication bias.
To our knowledge, this is a comprehensive systematic review and meta-analysis assessing the effectiveness and safety of SARS-CoV-2 vaccines based on real-world studies, reporting pooled VE for different variants of SARS-CoV-2 and incidence rate of adverse events. This meta-analysis comprised a total of 58 studies, including 32 studies for vaccine effectiveness and 26 studies for vaccine safety. We found that a single dose of SARS-CoV-2 vaccines was about 40–60% effective at preventing any clinical status of COVID-19 and that two doses were 85% or more effective. Although vaccines were not as effective against variants of SARS-CoV-2 as original virus, the vaccine effectiveness was still over 50% for fully vaccinated people. Normal adverse events were common, while the incidence of severe adverse events or even death was very low, providing reassurance to health care providers and to vaccine recipients and promote confidence in the safety of COVID-19 vaccines. Our findings strengthen and augment evidence from previous review [ 75 ], which confirmed the effectiveness of the BNT162b2 mRNA vaccine, and additionally reported the safety of SARS-CoV-2 vaccines, giving insight on the future of SARS-CoV-2 vaccine schedules.
Although most vaccines for the prevention of COVID-19 are two-dose vaccines, we found that the pooled VE of a single dose of SARS-CoV-2 vaccines was about 50%. Recent study showed that the T cell and antibody responses induced by a single dose of the BNT162b2 vaccine were comparable to those naturally infected with SARE-CoV-2 within weeks or months after infection [ 76 ]. Our findings could help to develop vaccination strategies under certain circumstances such as countries having a shortage of vaccines. In some countries, in order to administer the first dose to a larger population, the second dose was delayed for up to 12 weeks [ 77 ]. Some countries such as Canada had even decided to delay the second dose for 16 weeks [ 78 ]. However, due to a suboptimum immune response in those receiving only a single dose of a vaccine, such an approach had a chance to give rise to the emergence of variants of SARS-CoV-2 [ 79 ]. There remains a need for large clinical trials to assess the efficacy of a single-dose administration of two-dose vaccines and the risk of increasing the emergence of variants.
Two doses of SARS-CoV-2 vaccines were highly effective at preventing hospitalization, severe cases and deaths resulting from COVID-19, while the VE of different groups of days from the second vaccine dose showed no statistically significant differences. Our findings emphasized the importance of getting fully vaccinated, for the fact that most breakthrough infections were mild or asymptomatic. A recent study showed that the occurrence of breakthrough infections with SARS-CoV-2 in fully vaccinated populations was predictable with neutralizing antibody titers during the peri-infection period [ 80 ]. We also found getting fully vaccinated was at least 50% effective at preventing SARS-CoV-2 variants infections, despite reduced effectiveness compared with original virus; and BNT162b2 vaccine was found to have the highest VE in each variant group. Studies showed that the highly mutated variants were indicative of a form of rapid, multistage evolutionary jumps, which could preferentially occur in the milieu of partial immune control [ 81 , 82 ]. Therefore, immunocompromised patients should be prioritized for anti-COVID-19 immunization to mitigate persistent SARS-CoV-2 infections, during which multimutational SARS-CoV-2 variants could arise [ 83 ].
Recently, many countries, including Israel, the United States, China and the United Kingdom, have introduced a booster of COVID-19 vaccine, namely the third dose [ 84 , 85 , 86 , 87 ]. A study of Israel showed that among people vaccinated with BNT162b2 vaccine over 60 years, the risk of COVID-19 infection and severe illness in the non-booster group was 11.3 times (95% CI: 10.4–12.3) and 19.5 times (95% CI: 12.9–29.5) than the booster group, respectively [ 84 ]. Some studies have found that the third dose of Moderna, Pfizer-BioNTech, Oxford-AstraZeneca and Sinovac produced a spike in infection-blocking neutralizing antibodies when given a few months after the second dose [ 85 , 87 , 88 ]. In addition, the common adverse events associated with the third dose did not differ significantly from the symptoms of the first two doses, ranging from mild to moderate [ 85 ]. The overall incidence rate of local and systemic adverse events was 69% (57/97) and 20% (19/97) after receiving the third dose of BNT162b2 vaccine, respectively [ 88 ]. Results of a phase 3 clinical trial involving 306 people aged 18–55 years showed that adverse events after receiving a third dose of BNT162b2 vaccine (5–8 months after completion of two doses) were similar to those reported after receiving a second dose [ 85 ]. Based on V-safe, local reactions were more frequently after dose 3 (5323/6283; 84.7%) than dose 2 (5249/6283; 83.5%) among people who received 3 doses of Moderna. Systemic reactions were reported less frequently after dose 3 (4963/6283; 79.0%) than dose 2 (5105/6283; 81.3%) [ 86 ]. On August 4, WHO called for a halt to booster shots until at least the end of September to achieve an even distribution of the vaccine [ 89 ]. At this stage, the most important thing we should be thinking about is how to reach a global cover of people at risk with the first or second dose, rather than focusing on the third dose.
Based on real world studies, our results preliminarily showed that complete inoculation of COVID-19 vaccines was still effective against infection of variants, although the VE was generally diminished compared with the original virus. Particularly, the pooled VE was 54% (95% CI : 35–74%) for the Gamma variant, and 74% (95% CI : 62–85%) for the Delta variant. Since the wide spread of COVID-19, a number of variants have drawn extensive attention of international community, including Alpha variant (B.1.1.7), first identified in the United Kingdom; Beta variant (B.1.351) in South Africa; Gamma variant (P.1), initially appeared in Brazil; and the most infectious one to date, Delta variant (B.1.617.2) [ 90 ]. Israel recently reported a breakthrough infection of SARS-CoV-2, dominated by variant B.1.1.7 in a small number of fully vaccinated health care workers, raising concerns about the effectiveness of the original vaccine against those variants [ 80 ]. According to an observational cohort study in Qatar, VE of the BNT162b2 vaccine against the Alpha (B.1.1.7) and Beta (B.1.351) variants was 87% (95% CI : 81.8–90.7%) and 75.0% (95% CI : 70.5–7.9%), respectively [ 23 ]. Based on the National Immunization Management System of England, results from a recent real-world study of all the general population showed that the AZD1222 and BNT162b2 vaccines protected against symptomatic SARS-CoV-2 infection of Alpha variant with 74.5% (95% CI : 68.4–79.4%) and 93.7% (95% CI : 91.6–95.3%) [ 15 ]. In contrast, the VE against the Delta variant was 67.0% (95% CI : 61.3–71.8%) for two doses of AZD1222 vaccine and 88% (95% CI : 85.3–90.1%) for BNT162b2 vaccine [ 15 ].
In terms of adverse events after vaccination, the pooled incidence rate was very low, only 1.5% (95% CI : 1.4–1.6%). However, the prevalence of adverse events reported in large population (population size > 100 000) was much lower than that in small to medium population size. On the one hand, the vaccination population in the small to medium scale studies we included were mostly composed by health care workers, patients with specific diseases or the elderly. And these people are more concerned about their health and more sensitive to changes of themselves. But it remains to be proved whether patients or the elderly are more likely to have adverse events than the general. Mainstream vaccines currently on the market have maintained robust safety in specific populations such as cancer patients, organ transplant recipients, patients with rheumatic and musculoskeletal diseases, pregnant women and the elderly [ 54 , 91 , 92 , 93 , 94 ]. A prospective study by Tal Goshen-lag suggests that the safety of BNT162b2 vaccine in cancer patients is consistent with those previous reports [ 91 ]. In addition, the incidence rate of adverse events reported in the heart–lung transplant population is even lower than that in general population [ 95 ]. On the other hand, large scale studies at the national level are mostly based on national electronic health records or adverse event reporting systems, and it is likely that most mild or moderate symptoms are actually not reported.
Compared with the usual local adverse events (such as pain at the injection site, redness at the injection site, etc.) and normal systemic reactions (such as fatigue, myalgia, etc.), serious and life-threatening adverse events were rare due to our results. A meta-analysis based on RCTs only showed three cases of anaphylactic shock among 58 889 COVID-19 vaccine recipients and one in the placebo group [ 11 ]. The exact mechanisms underlying most of the adverse events are still unclear, accordingly we cannot establish a causal relation between severe adverse events and vaccination directly based on observational studies. In general, varying degrees of adverse events occur after different types of COVID-19 vaccination. Nevertheless, the benefits far outweigh the risks.
Our results showed the effectiveness and safety of different types of vaccines varied greatly. Regardless of SARS-CoV-2 variants, vaccine effectiveness varied from 66% (CoronaVac [ 14 ]) to 97% (mRNA-1273 [ 18 , 20 , 45 , 46 ]). The incidence rate of adverse events varied widely among different types of vaccines, which, however, could be explained by the sample size and population group of participants. BNT162b2, AZD1222, mRNA-1273 and CoronaVac were all found to have high vaccine efficacy and acceptable adverse-event profile in recent published studies [ 96 , 97 , 98 , 99 ]. A meta-analysis, focusing on the potential vaccine candidate which have reached to the phase 3 of clinical development, also found that although many of the vaccines caused more adverse events than the controls, most were mild, transient and manageable [ 100 ]. However, severe adverse events did occur, and there remains the need to implement a unified global surveillance system to monitor the adverse events of COVID-19 vaccines around the world [ 101 ]. A recent study employed a knowledge-based or rational strategy to perform a prioritization matrix of approved COVID-19 vaccines, and led to a scale with JANSSEN (Ad26.COV2.S) in the first place, and AZD1222, BNT162b2, and Sputnik V in second place, followed by BBIBP-CorV, CoronaVac and mRNA-1273 in third place [ 101 ]. Moreover, when deciding the priority of vaccines, the socioeconomic characteristics of each country should also be considered.
Our meta-analysis still has several limitations. First, we may include limited basic data on specific populations, as vaccination is slowly being promoted in populations under the age of 18 or over 60. Second, due to the limitation of the original real-world study, we did not conduct subgroup analysis based on more population characteristics, such as age. When analyzing the efficacy and safety of COVID-19 vaccine, we may have neglected the discussion on the heterogeneity from these sources. Third, most of the original studies only collected adverse events within 7 days after vaccination, which may limit the duration of follow-up for safety analysis.
Based on the real-world studies, SARS-CoV-2 vaccines have reassuring safety and could effectively reduce the death, severe cases, symptomatic cases, and infections resulting from SARS-CoV-2 across the world. In the context of global pandemic and the continuous emergence of SARS-CoV-2 variants, accelerating vaccination and improving vaccination coverage is still the most important and urgent matter, and it is also the final means to end the pandemic.
Availability of data and materials
All data generated or analyzed during this study are included in this published article and its additional information files.
Abbreviations
Coronavirus disease 2019
Severe Acute Respiratory Syndrome Coronavirus 2
Vaccine effectiveness
Confidence intervals
Intensive care unit
Random clinical trials
Preferred reporting items for systematic reviews and meta-analyses
COVID-19 Dashboard by the Center for Systems Science and Engineering (CSSE) at Johns Hopkins University (JHU). 2021. https://coronavirus.jhu.edu/map.html . Accessed 20 Aug 2021.
Barranco R, Rocca G, Molinelli A, Ventura F. Controversies and challenges of mass vaccination against SARS-CoV-2 in Italy: medico-legal perspectives and considerations. Healthcare (Basel). 2021. https://doi.org/10.3390/healthcare9091163 .
Article Google Scholar
COVID-19 vaccine tracker. 2021. https://vac-lshtm.shinyapps.io/ncov_vaccine_landscape/ . Accessed 20 Aug 2021.
Coronavirus (COVID-19) Vaccinations. 2021. https://ourworldindata.org/covid-vaccinations . Accessed 20 Aug 2021.
Kirby T. New variant of SARS-CoV-2 in UK causes surge of COVID-19. Lancet Respir Med. 2021;9(2):e20–1. https://doi.org/10.1016/s2213-2600(21)00005-9 .
Article CAS PubMed PubMed Central Google Scholar
Callaway E. Fast-spreading COVID variant can elude immune responses. Nature. 2021;589(7843):500–1. https://doi.org/10.1038/d41586-021-00121-z .
Article CAS PubMed Google Scholar
Reardon S. How the Delta variant achieves its ultrafast spread. Nature. 2021. https://doi.org/10.1038/d41586-021-01986-w .
Article PubMed Google Scholar
Li R, Liu J, Zhang H. The challenge of emerging SARS-CoV-2 mutants to vaccine development. J Genet Genomics. 2021;48(2):102–6. https://doi.org/10.1016/j.jgg.2021.03.001 .
Article PubMed PubMed Central Google Scholar
Chen M, Yuan Y, Zhou Y, Deng Z, Zhao J, Feng F, Zou H, Sun C. Safety of SARS-CoV-2 vaccines: a systematic review and meta-analysis of randomized controlled trials. Infect Dis Poverty. 2021;10(1):94. https://doi.org/10.1186/s40249-021-00878-5 .
Ling Y, Zhong J, Luo J. Safety and effectiveness of SARS-CoV-2 vaccines: a systematic review and meta-analysis. J Med Virol. 2021. https://doi.org/10.1002/jmv.27203 .
Pormohammad A, Zarei M, Ghorbani S, Mohammadi M, Razizadeh MH, Turner DL, Turner RJ. Efficacy and safety of COVID-19 vaccines: a systematic review and meta-analysis of randomized clinical trials. Vaccines (Basel). 2021. https://doi.org/10.3390/vaccines9050467 .
Sathian B, Asim M, Banerjee I, Roy B, Pizarro AB, Mancha MA, van Teijlingen ER, Kord-Varkaneh H, Mekkodathil AA, Subramanya SH, et al. Development and implementation of a potential coronavirus disease 2019 (COVID-19) vaccine: a systematic review and meta-analysis of vaccine clinical trials. Nepal J Epidemiol. 2021;11(1):959–82. https://doi.org/10.3126/nje.v11i1.36163 .
Yuan P, Ai P, Liu Y, Ai Z, Wang Y, Cao W, Xia X, Zheng JC. Safety, tolerability, and immunogenicity of COVID-19 vaccines: a systematic review and meta-analysis. medRxiv. 2020. https://doi.org/10.1101/2020.11.03.20224998 .
Jara A, Undurraga EA, González C, Paredes F, Fontecilla T, Jara G, Pizarro A, Acevedo J, Leo K, Leon F, et al. Effectiveness of an inactivated SARS-CoV-2 vaccine in Chile. N Engl J Med. 2021. https://doi.org/10.1056/NEJMoa2107715 .
Lopez Bernal J, Andrews N, Gower C, Gallagher E, Simmons R, Thelwall S, Stowe J, Tessier E, Groves N, Dabrera G, et al. Effectiveness of COVID-19 vaccines against the B.1.617.2 (Delta) variant. N Engl J Med. 2021. https://doi.org/10.1056/NEJMoa2108891 .
Israel says Pfizer Covid vaccine is just 39% effective as delta spreads, but still prevents severe illness. 2021. https://www.cnbc.com/2021/07/23/delta-variant-pfizer-covid-vaccine-39percent-effective-in-israel-prevents-severe-illness.html . Accessed 20 Aug 2021.
Zacay G, Shasha D, Bareket R, Kadim I, Hershkowitz Sikron F, Tsamir J, Mossinson D, Heymann AD. BNT162b2 vaccine effectiveness in preventing asymptomatic infection with SARS-CoV-2 virus: a nationwide historical cohort study. Open Forum Infect Dis. 2021;8(6): ofab262. https://doi.org/10.1093/ofid/ofab262 .
Martínez-Baz I, Miqueleiz A, Casado I, Navascués A, Trobajo-Sanmartín C, Burgui C, Guevara M, Ezpeleta C, Castilla J. Effectiveness of COVID-19 vaccines in preventing SARS-CoV-2 infection and hospitalisation, Navarre, Spain, January to April 2021. Eurosurveillance. 2021. https://doi.org/10.2807/1560-7917.Es.2021.26.21.2100438 .
Tenforde MW, Olson SM, Self WH, Talbot HK, Lindsell CJ, Steingrub JS, Shapiro NI, Ginde AA, Douin DJ, Prekker ME, et al. Effectiveness of Pfizer-BioNTech and moderna vaccines against COVID-19 among hospitalized adults aged ≥65 years—United States, January–March 2021. MMWR Morb Mortal Wkly Rep. 2021;70(18):674–9. https://doi.org/10.15585/mmwr.mm7018e1 .
Pawlowski C, Lenehan P, Puranik A, Agarwal V, Venkatakrishnan AJ, Niesen MJM, O’Horo JC, Virk A, Swift MD, Badley AD, et al. FDA-authorized mRNA COVID-19 vaccines are effective per real-world evidence synthesized across a multi-state health system. Med (N Y). 2021. https://doi.org/10.1016/j.medj.2021.06.007 .
Wells G, Shea B, O'Connell D, Peterson J, Welch V, Losos M, Tugwell P. The Newcastle-Ottawa Scale (NOS) for assessing the quality of nonrandomised studies in meta-analyses. http://www.ohri.ca/programs/clinical_epidemiology/oxford.asp . Accessed 20 Aug 2021.
Rostom A, Dubé C, Cranney A, et al. Celiac Disease. Rockville (MD): Agency for Healthcare Research and Quality (US); 2004 Sep. (Evidence Reports/Technology Assessments, No. 104.) Appendix D. Quality Assessment Forms. Available from: https://www.ncbi.nlm.nih.gov/books/NBK35156/ . Accessed 20 Aug 2021
Abu-Raddad LJ, Chemaitelly H, Butt AA. Effectiveness of the BNT162b2 COVID-19 vaccine against the B.1.1.7 and B.1.351 Variants. N Engl J Med. 2021;385(2):187–9. https://doi.org/10.1056/NEJMc2104974 .
Angel Y, Spitzer A, Henig O, Saiag E, Sprecher E, Padova H, Ben-Ami R. Association between vaccination with BNT162b2 and incidence of symptomatic and asymptomatic SARS-CoV-2 infections among health care workers. JAMA. 2021;325(24):2457–65. https://doi.org/10.1001/jama.2021.7152 .
Azamgarhi T, Hodgkinson M, Shah A, Skinner JA, Hauptmannova I, Briggs TWR, Warren S. BNT162b2 vaccine uptake and effectiveness in UK healthcare workers—a single centre cohort study. Nat Commun. 2021;12(1):3698. https://doi.org/10.1038/s41467-021-23927-x .
Bianchi FP, Germinario CA, Migliore G, Vimercati L, Martinelli A, Lobifaro A, Tafuri S, Stefanizzi P. BNT162b2 mRNA COVID-19 vaccine effectiveness in the prevention of SARS-CoV-2 infection: a preliminary report. J Infect Dis. 2021. https://doi.org/10.1093/infdis/jiab262 .
Britton A, Jacobs Slifka KM, Edens C, Nanduri SA, Bart SM, Shang N, Harizaj A, Armstrong J, Xu K, Ehrlich HY, et al. Effectiveness of the Pfizer-BioNTech COVID-19 vaccine among residents of two skilled nursing facilities experiencing COVID-19 outbreaks—Connecticut, December 2020–February 2021. MMWR Morb Mortal Wkly Rep. 2021;70(11):396–401. https://doi.org/10.15585/mmwr.mm7011e3 .
Cavanaugh AM, Fortier S, Lewis P, Arora V, Johnson M, George K, Tobias J, Lunn S, Miller T, Thoroughman D, et al. COVID-19 outbreak associated with a SARS-CoV-2 R1 lineage variant in a skilled nursing facility after vaccination program—Kentucky, March 2021. MMWR Morb Mortal Wkly Rep. 2021;70(17):639–43. https://doi.org/10.15585/mmwr.mm7017e2 .
Chemaitelly H, Yassine HM, Benslimane FM, Al Khatib HA, Tang P, Hasan MR, Malek JA, Coyle P, Ayoub HH, Al Kanaani Z, et al. mRNA-1273 COVID-19 vaccine effectiveness against the B.1.1.7 and B.1.351 variants and severe COVID-19 disease in Qatar. Nat Med. 2021. https://doi.org/10.1038/s41591-021-01446-y .
Chodick G, Tene L, Patalon T, Gazit S, Ben Tov A, Cohen D, Muhsen K. Assessment of effectiveness of 1 dose of BNT162b2 vaccine for SARS-CoV-2 infection 13 to 24 days after immunization. JAMA Netw Open. 2021;4(6): e2115985. https://doi.org/10.1001/jamanetworkopen.2021.15985 .
Chodick G, Tene L, Rotem RS, Patalon T, Gazit S, Ben-Tov A, Weil C, Goldshtein I, Twig G, Cohen D, et al. The effectiveness of the TWO-DOSE BNT162b2 vaccine: analysis of real-world data. Clin Infect Dis. 2021. https://doi.org/10.1093/cid/ciab438 .
Dagan N, Barda N, Kepten E, Miron O, Perchik S, Katz MA, Hernán MA, Lipsitch M, Reis B, Balicer RD. BNT162b2 mRNA COVID-19 vaccine in a nationwide mass vaccination setting. N Engl J Med. 2021;384(15):1412–23. https://doi.org/10.1056/NEJMoa2101765 .
Flacco ME, Soldato G, Acuti Martellucci C, Carota R, Di Luzio R, Caponetti A, Manzoli L. Interim estimates of COVID-19 vaccine effectiveness in a mass vaccination setting: data from an Italian Province. VacCInes (Basel). 2021. https://doi.org/10.3390/vaccines9060628 .
Haas EJ, Angulo FJ, McLaughlin JM, Anis E, Singer SR, Khan F, Brooks N, Smaja M, Mircus G, Pan K, et al. Impact and effectiveness of mRNA BNT162b2 vaccine against SARS-CoV-2 infections and COVID-19 cases, hospitalisations, and deaths following a nationwide vaccination campaign in Israel: an observational study using national surveillance data. Lancet. 2021;397(10287):1819–29. https://doi.org/10.1016/s0140-6736(21)00947-8 .
Hall VJ, Foulkes S, Saei A, Andrews N, Oguti B, Charlett A, Wellington E, Stowe J, Gillson N, Atti A, et al. COVID-19 vaccine coverage in health-care workers in England and effectiveness of BNT162b2 mRNA vaccine against infection (SIREN): a prospective, multicentre, cohort study. Lancet. 2021;397(10286):1725–35. https://doi.org/10.1016/s0140-6736(21)00790-x .
Hyams C, Marlow R, Maseko Z, King J, Ward L, Fox K, Heath R, Tuner A, Friedrich Z, Morrison L, et al. Effectiveness of BNT162b2 and ChAdOx1 nCoV-19 COVID-19 vaccination at preventing hospitalisations in people aged at least 80 years: a test-negative, case-control study. Lancet Infect Dis. 2021. https://doi.org/10.1016/s1473-3099(21)00330-3 .
Khan N, Mahmud N. Effectiveness of SARS-CoV-2 vaccination in a veterans affairs cohort of patients with inflammatory bowel disease with diverse exposure to immunosuppressive medications. Gastroenterology. 2021. https://doi.org/10.1053/j.gastro.2021.05.044 .
Knobel P, Serra C, Grau S, Ibañez R, Diaz P, Ferrández O, Villar R, Lopez AF, Pujolar N, Horcajada JP, et al. COVID-19 mRNA vaccine effectiveness in asymptomatic healthcare workers. Infect Control Hosp Epidemiol. 2021. https://doi.org/10.1017/ice.2021.287 .
Lopez Bernal J, Andrews N, Gower C, Robertson C, Stowe J, Tessier E, Simmons R, Cottrell S, Roberts R, O’Doherty M, et al. Effectiveness of the Pfizer-BioNTech and Oxford-AstraZeneca vaccines on covid-19 related symptoms, hospital admissions, and mortality in older adults in England: test negative case-control study. BMJ. 2021;373: n1088. https://doi.org/10.1136/bmj.n1088 .
Mazagatos C, Monge S, Olmedo C, Vega L, Gallego P, Martín-Merino E, Sierra MJ, Limia A, Larrauri A. Effectiveness of mRNA COVID-19 vaccines in preventing SARS-CoV-2 infections and COVID-19 hospitalisations and deaths in elderly long-term care facility residents, Spain, weeks 53, 2020 to 13 2021. Eurosurveillance. 2021. https://doi.org/10.2807/1560-7917.Es.2021.26.24.2100452 .
Pilishvili T, Fleming-Dutra KE, Farrar JL, Gierke R, Mohr NM, Talan DA, Krishnadasan A, Harland KK, Smithline HA, Hou PC, et al. Interim estimates of vaccine effectiveness of Pfizer-BioNTech and Moderna COVID-19 vaccines among health care personnel—33 US Sites, January–March 2021. MMWR Morb Mortal Wkly Rep. 2021;70(20):753–8. https://doi.org/10.15585/mmwr.mm7020e2 .
Sheikh A, McMenamin J, Taylor B, Robertson C. SARS-CoV-2 Delta VOC in Scotland: demographics, risk of hospital admission, and vaccine effectiveness. Lancet. 2021;397(10293):2461–2. https://doi.org/10.1016/s0140-6736(21)01358-1 .
Shrotri M, Krutikov M, Palmer T, Giddings R, Azmi B, Subbarao S, Fuller C, Irwin-Singer A, Davies D, Tut G, et al. Vaccine effectiveness of the first dose of ChAdOx1 nCoV-19 and BNT162b2 against SARS-CoV-2 infection in residents of long-term care facilities in England (VIVALDI): a prospective cohort study. Lancet Infect Dis. 2021. https://doi.org/10.1016/s1473-3099(21)00289-9 .
Skowronski DM, Setayeshgar S, Zou M, Prystajecky N, Tyson JR, Galanis E, Naus M, Patrick DM, Sbihi H, El Adam S, et al. Single-dose mRNA vaccine effectiveness against SARS-CoV-2, including Alpha and Gamma variants: a test-negative design in adults 70 years and older in British Columbia,Canada. Clin Infect Dis. 2021. https://doi.org/10.1093/cid/ciab616 .
Swift MD, Breeher LE, Tande AJ, Tommaso CP, Hainy CM, Chu H, Murad MH, Berbari EF, Virk A. Effectiveness of mRNA COVID-19 vaccines against SARS-CoV-2 infection in a cohort of healthcare personnel. Clin Infect Dis. 2021. https://doi.org/10.1093/cid/ciab361 .
Thompson MG, Burgess JL, Naleway AL, Tyner H, Yoon SK, Meece J, Olsho LEW, Caban-Martinez AJ, Fowlkes AL, Lutrick K, et al. Prevention and attenuation of COVID-19 with the BNT162b2 and mRNA-1273 Vaccines. N Engl J Med. 2021. https://doi.org/10.1056/NEJMoa2107058 .
Vasileiou E, Simpson CR, Shi T, Kerr S, Agrawal U, Akbari A, Bedston S, Beggs J, Bradley D, Chuter A, et al. Interim findings from first-dose mass COVID-19 vaccination roll-out and COVID-19 hospital admissions in Scotland: a national prospective cohort study. Lancet. 2021;397(10285):1646–57. https://doi.org/10.1016/s0140-6736(21)00677-2 .
Williams C, Al-Bargash D, Macalintal C, Stuart R, Seth A, Latham J, Gitterman L, Fedsin S, Godoy M, Kozak R, et al. COVID-19 outbreak associated with a SARS-CoV-2 P.1 lineage in a long-term care home after implementation of a vaccination program—Ontario, April–May 2021. Clin Infect Dis. 2021. https://doi.org/10.1093/cid/ciab617 .
Alhazmi A, Alamer E, Daws D, Hakami M, Darraj M, Abdelwahab S, Maghfuri A, Algaissi A. Evaluation of side effects associated with COVID-19 vaccines in Saudi Arabia. Vaccines (Basel). 2021. https://doi.org/10.3390/vaccines9060674 .
Andrzejczak-Grządko S, Czudy Z, Donderska M. Side effects after COVID-19 vaccinations among residents of Poland. Eur Rev Med Pharmacol Sci. 2021;25(12):4418–21. https://doi.org/10.26355/eurrev_202106_26153 .
Baldolli A, Michon J, Appia F, Galimard C, Verdon R, Parienti JJ. Tolerance of BNT162b2 mRNA COVI-19 vaccine in patients with a medical history of COVID-19 disease: a case control study. Vaccine. 2021;39(32):4410–3. https://doi.org/10.1016/j.vaccine.2021.06.054 .
Cherian S, Paul A, Ahmed S, Alias B, Manoj M, Santhosh AK, Varghese DR, Krishnan N, Shenoy P. Safety of the ChAdOx1 nCoV-19 and the BBV152 vaccines in 724 patients with rheumatic diseases: a post-vaccination cross-sectional survey. Rheumatol Int. 2021;41(8):1441–5. https://doi.org/10.1007/s00296-021-04917-0 .
Chevallier P, Coste-Burel M, Le Bourgeois A, Peterlin P, Garnier A, Béné MC, Imbert BM, Drumel T, Le Gouill S, Moreau P, et al. Safety and immunogenicity of a first dose of SARS-CoV-2 mRNA vaccine in allogeneic hematopoietic stem-cells recipients. EJHaem. 2021. https://doi.org/10.1002/jha2.242 .
Connolly CM, Ruddy JA, Boyarsky BJ, Avery RK, Werbel WA, Segev DL, Garonzik-Wang J, Paik JJ. Safety of the first dose of mRNA SARS-CoV-2 vaccines in patients with rheumatic and musculoskeletal diseases. Ann Rheum Dis. 2021. https://doi.org/10.1136/annrheumdis-2021-220231 .
Furer V, Eviatar T, Zisman D, Peleg H, Paran D, Levartovsky D, Zisapel M, Elalouf O, Kaufman I, Meidan R, et al. Immunogenicity and safety of the BNT162b2 mRNA COVID-19 vaccine in adult patients with autoimmune inflammatory rheumatic diseases and in the general population: a multicentre study. Ann Rheum Dis. 2021. https://doi.org/10.1136/annrheumdis-2021-220647 .
Gee J, Marquez P, Su J, Calvert GM, Liu R, Myers T, Nair N, Martin S, Clark T, Markowitz L, et al. First month of COVID-19 vaccine safety monitoring—United States, December 14, 2020–January 13, 2021. MMWR Morb Mortal Wkly Rep. 2021;70(8):283–8. https://doi.org/10.15585/mmwr.mm7008e3 .
Hashimoto T, Ozaki A, Bhandari D, Sawano T, Sah R, Tanimoto T. High anaphylaxis rates following vaccination with the Pfizer BNT162b2 mRNA vaccine against COVID-19 in Japanese health care workers; a secondary analysis of initial post-approval safety data. J Travel Med. 2021. https://doi.org/10.1093/jtm/taab090 .
Lv G, Yuan J, Xiong X, Li M. Mortality rate and characteristics of deaths following COVID-19 vaccination. Front Med (Lausanne). 2021;8: 670370. https://doi.org/10.3389/fmed.2021.670370 .
McMurry R, Lenehan P, Awasthi S, Silvert E, Puranik A, Pawlowski C, Venkatakrishnan AJ, Anand P, Agarwal V, O’Horo JC, et al. Real-time analysis of a mass vaccination effort confirms the safety of FDA-authorized mRNA COVID-19 vaccines. Med (N Y). 2021. https://doi.org/10.1016/j.medj.2021.06.006 .
Monin L, Laing AG, Muñoz-Ruiz M, McKenzie DR, Del Molino Del Barrio I, Alaguthurai T, Domingo-Vila C, Hayday TS, Graham C, Seow J, et al. Safety and immunogenicity of one versus two doses of the COVID-19 vaccine BNT162b2 for patients with cancer: interim analysis of a prospective observational study. Lancet Oncol. 2021;22(6):765–78. https://doi.org/10.1016/s1470-2045(21)00213-8 .
Pagotto V, Ferloni A, Mercedes Soriano M, Díaz M, Braguinsky Golde N, González MI, Asprea V, Staneloni MI, Zingoni P, Vidal G, et al. Active monitoring of early safety of Sputnik V vaccine in Buenos Aires, Argentina. MediCIna (B Aires). 2021;81(3):408–14.
Google Scholar
Peled Y, Ram E, Lavee J, Sternik L, Segev A, Wieder-Finesod A, Mandelboim M, Indenbaum V, Levy I, Raanani E, et al. BNT162b2 vaccination in heart transplant recipients: Clinical experience and antibody response. J Heart Lung Transplant. 2021. https://doi.org/10.1016/j.healun.2021.04.003 .
Quiroga B, Sánchez-Álvarez E, Goicoechea M, de Sequera P. COVID-19 vaccination among Spanish nephrologists: acceptance and side effects. J Healthc Qual Res. 2021. https://doi.org/10.1016/j.jhqr.2021.05.002 .
Ram R, Hagin D, Kikozashvilli N, Freund T, Amit O, Bar-On Y, Beyar-Katz O, Shefer G, Moshiashvili MM, Karni C, et al. Safety and immunogenicity of the BNT162b2 mRNA COVID-19 vaccine in patients after allogeneic HCT or CD19-based CART therapy—a single center prospective cohort study. Transplant Cell Ther. 2021. https://doi.org/10.1016/j.jtct.2021.06.024 .
Revon-Riviere G, Ninove L, Min V, Rome A, Coze C, Verschuur A, de Lamballerie X, André N. The BNT162b2 mRNA COVID-19 vaccine in adolescents and young adults with cancer: a monocentric experience. Eur J Cancer. 2021;154:30–4. https://doi.org/10.1016/j.ejca.2021.06.002 .
Riad A, Pokorná A, Mekhemar M, Conrad J, Klugarová J, Koščík M, Klugar M, Attia S. Safety of ChAdOx1 nCoV-19 vaccine: independent evidence from two EU states. Vaccines (Basel). 2021. https://doi.org/10.3390/vaccines9060673 .
Riad A, Sağıroğlu D, Üstün B, Pokorná A, Klugarová J, Attia S, Klugar M. Prevalence and risk factors of CoronaVac Side effects: an independent cross-sectional study among healthcare workers in Turkey. J Clin Med. 2021. https://doi.org/10.3390/jcm10122629 .
Rosman Y, Lavi N, Meir-Shafrir K, Lachover-Roth I, Cohen-Engler A, Mekori YA, Confino-Cohen R. Safety of BNT162b2 mRNA COVID-19 vaccine in patients with mast cell disorders. J Allergy Clin Immunol Pract. 2021. https://doi.org/10.1016/j.jaip.2021.06.032 .
Signorelli C, Odone A, Gianfredi V, Capraro M, Kacerik E, Chiecca G, Scardoni A, Minerva M, Mantecca R, Musarò P, et al. Application of the “immunization islands” model to improve quality, efficiency and safety of a COVID-19 mass vaccination site. Ann Ig. 2021;33(5):499–512. https://doi.org/10.7416/ai.2021.2456 .
Vallée A, Chan-Hew-Wai A, Bonan B, Lesprit P, Parquin F, Catherinot É, Choucair J, Billard D, Amiel-Taieb C, Camps È, et al. Oxford-AstraZeneca COVID-19 vaccine: need of a reasoned and effective vaccine campaign. Public Health. 2021;196:135–7. https://doi.org/10.1016/j.puhe.2021.05.030 .
Wang J, Hou Z, Liu J, Gu Y, Wu Y, Chen Z, Ji J, Diao S, Qiu Y, Zou S, et al. Safety and immunogenicity of COVID-19 vaccination in patients with non-alcoholic fatty liver disease (CHESS2101): a multicenter study. J Hepatol. 2021. https://doi.org/10.1016/j.jhep.2021.04.026 .
Zhang MX, Zhang TT, Shi GF, Cheng FM, Zheng YM, Tung TH, Chen HX. Safety of an inactivated SARS-CoV-2 vaccine among healthcare workers in China. Expert Rev Vaccines. 2021. https://doi.org/10.1080/14760584.2021.1925112 .
Shay DK, Gee J, Su JR, Myers TR, Marquez P, Liu R, Zhang B, Licata C, Clark TA, Shimabukuro TT. Safety monitoring of the Janssen (Johnson & Johnson) COVID-19 Vaccine—United States, March–April 2021. MMWR Morb Mortal Wkly Rep. 2021;70(18):680–4. https://doi.org/10.15585/mmwr.mm7018e2 .
Prevention CCfDCa. Information analysis of COVID-19 vaccine adverse reaction monitoring in China. 2021-5-28. http://www.chinacdc.cn/jkzt/ymyjz/ymyjjz_6758/202105/t20210528_230908.html . Accessed 20 Aug 2021.
Kow CS, Hasan SS. Real-world effectiveness of BNT162b2 mRNA vaccine: a meta-analysis of large observational studies. Inflammopharmacology. 2021;29(4):1075–90. https://doi.org/10.1007/s10787-021-00839-2 .
Angyal A, Longet S, Moore S, Payne RP, Harding A et al. T-Cell and Antibody Responses to First BNT162b2 Vaccine Dose in Previously SARS-CoV-2-Infected and Infection-Naive UK Healthcare Workers: A Multicentre, Prospective, Observational Cohort Study. Available at SSRN: https://ssrn.com/abstract=3820576 or https://doi.org/10.2139/ssrn.3820576 . Accessed 20 Aug 2021.
Pimenta D, Yates C, Pagel C, Gurdasani D. Delaying the second dose of covid-19 vaccines. BMJ. 2021;372: n710. https://doi.org/10.1136/bmj.n710 .
Tauh T, Mozel M, Meyler P, Lee SM. An updated look at the 16-week window between doses of vaccines in BC for COVID-19. BC Med J. 2021;63(3):102–3.
Kadire SR, Wachter RM, Lurie N. Delayed second dose versus standard regimen for COVID-19 vaccination. N Engl J Med. 2021;384(9): e28. https://doi.org/10.1056/NEJMclde2101987 .
Bergwerk M, Gonen T, Lustig Y, Amit S, Lipsitch M, Cohen C, Mandelboim M, Gal Levin E, Rubin C, Indenbaum V, et al. COVID-19 breakthrough infections in vaccinated health care workers. N Engl J Med. 2021. https://doi.org/10.1056/NEJMoa2109072 .
Truong TT, Ryutov A, Pandey U, Yee R, Goldberg L, Bhojwani D, Aguayo-Hiraldo P, Pinsky BA, Pekosz A, Shen L, et al. Persistent SARS-CoV-2 infection and increasing viral variants in children and young adults with impaired humoral immunity. medRxiv. 2021. https://doi.org/10.1101/2021.02.27.21252099 .
Choi B, Choudhary MC, Regan J, Sparks JA, Padera RF, Qiu X, Solomon IH, Kuo HH, Boucau J, Bowman K, et al. Persistence and evolution of SARS-CoV-2 in an Immunocompromised Host. N Engl J Med. 2020;383(23):2291–3. https://doi.org/10.1056/NEJMc2031364 .
Corey L, Beyrer C, Cohen MS, Michael NL, Bedford T, Rolland M. SARS-CoV-2 variants in patients with immunosuppression. N Engl J Med. 2021;385(6):562–6. https://doi.org/10.1056/NEJMsb2104756 .
Bar-On YM, Goldberg Y, Mandel M, Bodenheimer O, Freedman L, Kalkstein N, Mizrahi B, Alroy-Preis S, Ash N, Milo R, et al. Protection of BNT162b2 vaccine booster against Covid-19 in Israel. N Engl J Med. 2021;385(15):1393–400. https://doi.org/10.1056/NEJMoa2114255 .
Hause AM, Baggs J, Gee J, Marquez P, Myers TR, Shimabukuro TT, Shay DK. Safety monitoring of an additional dose of COVID-19 vaccine—United States, August 12–September 19, 2021. MMWR Morb Mortal Wkly Rep. 2021;70(39):1379–84. https://doi.org/10.15585/mmwr.mm7039e4 .
Furlow B. Immunocompromised patients in the USA and UK should receive third dose of COVID-19 vaccine. Lancet Rheumatol. 2021. https://doi.org/10.1016/s2665-9913(21)00313-1 .
Flaxman A, Marchevsky NG, Jenkin D, Aboagye J, Aley PK, Angus B, Belij-Rammerstorfer S, Bibi S, Bittaye M, Cappuccini F, et al. Reactogenicity and immunogenicity after a late second dose or a third dose of ChAdOx1 nCoV-19 in the UK: a substudy of two randomised controlled trials (COV001 and COV002). Lancet. 2021;398(10304):981–90. https://doi.org/10.1016/s0140-6736(21)01699-8 .
Peled Y, Ram E, Lavee J, Segev A, Matezki S, Wieder-Finesod A, Halperin R, Mandelboim M, Indenbaum V, Levy I, et al. Third dose of the BNT162b2 vaccine in heart transplant recipients: immunogenicity and clinical experience. J Heart Lung Transplant. 2021. https://doi.org/10.1016/j.healun.2021.08.010 .
WHO. WHO press conference on coronavirus disease (COVID-19)—4 August 2021. 2021. https://www.who.int/multi-media/details/who-press-conference-on-coronavirus-disease-(covid-19)---4-august-2021 . Accessed 20 Aug 2021.
Cascella M, Rajnik M, Aleem A, Dulebohn SC, Di Napoli R. Features, evaluation, and treatment of coronavirus (COVID-19). In: StatPearls. edn. Treasure Island (FL): StatPearls Publishing Copyright © 2021, StatPearls Publishing LLC.; 2021.
Goshen-Lago T, Waldhorn I, Holland R, Szwarcwort-Cohen M, Reiner-Benaim A, Shachor-Meyouhas Y, Hussein K, Fahoum L, Baruch M, Peer A, et al. Serologic status and toxic effects of the SARS-CoV-2 BNT162b2 vaccine in patients undergoing treatment for cancer. JAMA Oncol. 2021. https://doi.org/10.1001/jamaoncol.2021.2675 .
Ou MT, Boyarsky BJ, Motter JD, Greenberg RS, Teles AT, Ruddy JA, Krach MR, Jain VS, Werbel WA, Avery RK, et al. Safety and reactogenicity of 2 doses of SARS-CoV-2 vaccination in solid organ transplant recipients. Transplantation. 2021. https://doi.org/10.1097/tp.0000000000003780 .
Bookstein Peretz S, Regev N, Novick L, Nachshol M, Goffer E, Ben-David A, Asraf K, Doolman R, Sapir E, Regev Yochay G, et al. Short-term outcome of pregnant women vaccinated by BNT162b2 mRNA COVID-19 vaccine. Ultrasound Obstet Gynecol. 2021. https://doi.org/10.1002/uog.23729 .
Shimabukuro TT, Kim SY, Myers TR, Moro PL, Oduyebo T, Panagiotakopoulos L, Marquez PL, Olson CK, Liu R, Chang KT, et al. Preliminary findings of mRNA COVID-19 vaccine safety in pregnant persons. N Engl J Med. 2021;384(24):2273–82. https://doi.org/10.1056/NEJMoa2104983 .
Peled Y, Ram E, Lavee J, Sternik L, Segev A, Wieder-Finesod A, Mandelboim M, Indenbaum V, Levy I, Raanani E, et al. BNT162b2 vaccination in heart transplant recipients: clinical experience and antibody response. J Heart Lung Transplant. 2021;40(8):759–62. https://doi.org/10.1016/j.healun.2021.04.003 .
Thomas SJ, Moreira ED Jr, Kitchin N, Absalon J, Gurtman A, Lockhart S, Perez JL, Pérez Marc G, Polack FP, Zerbini C, et al. Safety and efficacy of the BNT162b2 mRNA COVID-19 vaccine through 6 months. N Engl J Med. 2021. https://doi.org/10.1056/NEJMoa2110345 .
Falsey AR, Sobieszczyk ME, Hirsch I, Sproule S, Robb ML, Corey L, Neuzil KM, Hahn W, Hunt J, Mulligan MJ, et al. Phase 3 safety and efficacy of AZD1222 (ChAdOx1 nCoV-19) COVID-19 vaccine. N Engl J Med. 2021. https://doi.org/10.1056/NEJMoa2105290 .
El Sahly HM, Baden LR, Essink B, Doblecki-Lewis S, Martin JM, Anderson EJ, Campbell TB, Clark J, Jackson LA, Fichtenbaum CJ, et al. Efficacy of the mRNA-1273 SARS-CoV-2 vaccine at completion of blinded phase. N Engl J Med. 2021. https://doi.org/10.1056/NEJMoa2113017 .
Tanriover MD, Doğanay HL, Akova M, Güner HR, Azap A, Akhan S, Köse Ş, Erdinç F, Akalın EH, Tabak ÖF, et al. Efficacy and safety of an inactivated whole-virion SARS-CoV-2 vaccine (CoronaVac): interim results of a double-blind, randomised, placebo-controlled, phase 3 trial in Turkey. Lancet. 2021;398(10296):213–22. https://doi.org/10.1016/s0140-6736(21)01429-x .
Kumar S, Saurabh MK, Maharshi V. Efficacy and safety of potential vaccine candidates against coronavirus disease 2019: a systematic review. J Adv Pharm Technol Res. 2021;12(3):215–21. https://doi.org/10.4103/japtr.JAPTR_229_20 .
Burgos-Salcedo J. A rational strategy to support approved COVID-19 vaccines prioritization. Hum Vaccin Immunother. 2021;17(10):3474–7. https://doi.org/10.1080/21645515.2021.1922060 .
Download references
Acknowledgements
This study was funded by the National Natural Science Foundation of China (72122001; 71934002) and the National Science and Technology Key Projects on Prevention and Treatment of Major infectious disease of China (2020ZX10001002). The funders had no role in study design, data collection and analysis, decision to publish, or preparation of the paper. No payment was received by any of the co-authors for the preparation of this article.
Author information
Qiao Liu and Chenyuan Qin are joint first authors
Authors and Affiliations
Department of Epidemiology and Biostatistics, School of Public Health, Peking University, Beijing, 100191, China
Qiao Liu, Chenyuan Qin, Min Liu & Jue Liu
Institute for Global Health and Development, Peking University, Beijing, 100871, China
Chenyuan Qin & Jue Liu
You can also search for this author in PubMed Google Scholar
Contributions
LQ and QCY contributed equally as first authors. LJ and LM contributed equally as correspondence authors. LJ and LM conceived and designed the study; LQ, QCY and LJ carried out the literature searches, extracted the data, and assessed the study quality; LQ and QCY performed the statistical analysis and wrote the manuscript; LJ, LM, LQ and QCY revised the manuscript. All authors read and approved the final manuscript.
Corresponding authors
Correspondence to Min Liu or Jue Liu .
Ethics declarations
Ethics approval and consent to participate.
Not applicable.
Consent for publication
Competing interests.
The authors have no conflicts of interest to declare that are relevant to the content of this article.
Supplementary Information
Additional file 1: table s1..
Characteristic of studies included for vaccine effectiveness.
Additional file 2: Table S2.
Characteristic of studies included for vaccine safety.
Rights and permissions
Open Access This article is licensed under a Creative Commons Attribution 4.0 International License, which permits use, sharing, adaptation, distribution and reproduction in any medium or format, as long as you give appropriate credit to the original author(s) and the source, provide a link to the Creative Commons licence, and indicate if changes were made. The images or other third party material in this article are included in the article's Creative Commons licence, unless indicated otherwise in a credit line to the material. If material is not included in the article's Creative Commons licence and your intended use is not permitted by statutory regulation or exceeds the permitted use, you will need to obtain permission directly from the copyright holder. To view a copy of this licence, visit http://creativecommons.org/licenses/by/4.0/ . The Creative Commons Public Domain Dedication waiver ( http://creativecommons.org/publicdomain/zero/1.0/ ) applies to the data made available in this article, unless otherwise stated in a credit line to the data.
Reprints and permissions
About this article
Cite this article.
Liu, Q., Qin, C., Liu, M. et al. Effectiveness and safety of SARS-CoV-2 vaccine in real-world studies: a systematic review and meta-analysis. Infect Dis Poverty 10 , 132 (2021). https://doi.org/10.1186/s40249-021-00915-3
Download citation
Received : 07 September 2021
Accepted : 01 November 2021
Published : 14 November 2021
DOI : https://doi.org/10.1186/s40249-021-00915-3
Share this article
Anyone you share the following link with will be able to read this content:
Sorry, a shareable link is not currently available for this article.
Provided by the Springer Nature SharedIt content-sharing initiative
- Effectiveness
- Meta-analysis
Infectious Diseases of Poverty
ISSN: 2049-9957
- Submission enquiries: Access here and click Contact Us
- General enquiries: [email protected]
SYSTEMATIC REVIEW article
How has research on the effectiveness and safety of covid-19 vaccination been evaluated: a scope review with emphasis on coronavac.
- 1 Grupo de Epidemiología, Universidad de Antioquia, Medellín, Colombia
- 2 Grupo de Enfermedades Tropicales y Resistencia Bacteriana, Universidad del Sinú, Montería, Colombia
- 3 Grupo de investigación EMAP - Estadística y Matemáticas Aplicadas, Pontificia Universidad Javeriana, Cali, Colombia
- 4 Grupo de Investigación, Secretaría de Salud Distrital, Cali, Colombia
- 5 Grupo de Investigación Clínica - PECET (GIC-PECET), Universidad de Antioquia, Medellín, Colombia
- 6 Grupo de Investigación en Economía, Gestión y Salud, ECGESA. Pontificia Universidad Javeriana, Cali, Colombia
- 7 Departamento de Salud pública y Epidemiología, Pontificia Universidad Javeriana, Cali, Colombia
Introduction: The control of the COVID-19 epidemic has been focused on the development of vaccines against SARS-CoV-2. All developed vaccines have reported safety and efficacy results in preventing infection and its consequences, although the quality of evidence varies depending on the vaccine considered. Different methodological designs have been used for their evaluation, which can influence our understanding of the effects of these interventions. CoronaVac is an inactivated vaccine, and it has been assessed in various studies, including clinical trials and observational studies. Given these differences, our objective was to explore the published information to answer the question: how has the efficacy/effectiveness and safety of CoronaVac been evaluated in different studies? This is to identify potential gaps and challenges to be addressed in understanding its effect.
Methods: A scoping review was carried out following the methodology proposed by the Joanna Briggs Institute, which included studies carried out in humans as of 2020, corresponding to systematic reviews, clinical trials, analytical or descriptive observational studies, in which the effectiveness and/or safety of vaccines for COVID19 were evaluated or described. There were no age restrictions for the study participants.
Results: The efficacy/effectiveness and safety of this vaccine was assessed through 113 studies. Nineteen corresponded to experimental studies, 7 of Phase II, 5 of Phase IV, and 4 were clinical trials with random assignment. Although some clinical trials with random assignment have been carried out, these have limitations in terms of feasibility, follow-up times, and with this, the possibility of evaluating safety outcomes that occur with low frequencies. Not all studies have used homogeneous methods of analysis. Both the prevention of infection, and the prevention of outcomes such as hospitalization or death, have been valued through similar outcomes, but some through multivariate analysis of dependencies, and others through analysis that try to infer causally through different control methods of confounding.
Conclusion: Published information on the evaluation of the efficacy/effectiveness and safety of the CoronaVac is abundant. However, there are differences in terms of vaccine application schedules, population definition, outcomes evaluated, follow-up times, and safety assessment, as well as non-standardization in the reporting of results, which may hinder the generalizability of the findings. It is important to generate meetings and consensus strategies for the methods and reporting of this type of studies, which will allow to reduce the heterogeneity in their presentation and a better understanding of the effect of these vaccines.
1 Introduction
Starting from the first reports coming from China and from countries in Europe and Asia, about the infection produced by SARS-CoV-2, its high contagion, and lethality of up to 14% in older adults, and the subsequent declaration of a COVID-19 pandemic, and together with the measures established by the healthcare authorities to manage the disease, efforts began to develop effective and safe vaccines that would contribute to speeding up the control of this health condition, through the reduction of infections, complications, and deaths associated with this disease ( 1 ).
For this reason, pandemic control efforts have focused on developing vaccines against SARS-CoV-2 that are capable of acting against infection, disease, or transmission, and thus contribute to disease control ( 2 ). In this context, different research groups have developed vaccines using different platforms, including mRNA, viral vectors, and inactivated viruses ( 3 ).
Unlike most drugs, whose benefits are limited to the individual taking them, vaccines have the potential to produce far-reaching effects on general public health and well-being, cognitive development, and, ultimately, economic productivity ( 4 ). However, the global advances in vaccination coverage achieved during the first years of the 21st century have been threatened by the emergence of anti-vaccination groups that have questioned vaccine efficacy to create public distrust of vaccines and immunization programs. This requires an adequate and conscious evaluation of both the efficacy/effectiveness and the different aspects that can affect the safety of the people who receive them ( 5 ).
In general, vaccines that have gained approval for human use have been effective in preventing COVID-19, particularly in preventing severe disease and death. However, reports on their implementation are mainly based on follow-up studies of the adult population ( 6 ). Additionally, if the vaccination prevents symptoms from developing and asymptomatic infections are less likely to be discovered than symptomatic ones, it is feasible that the effectiveness against any infection has been overstated. A competitive tendency toward underestimate arises when estimates are based on tests with inadequate specificity, particularly when testing are conducted more frequently than has been estimated for various COVID-19 vaccinations ( 7 ).
All vaccines seem to be safe and efficacious against all variations of interest in preventing hospitalization, death, and severe COVID-19; however, the quality of the data differs significantly between the vaccines under consideration ( 8 ).
Different methodological designs have been used to evaluate the effectiveness and safety of vaccines for COVID-19. Most clinical trials were carried out before the appearance of variants of concern, and the duration, subgroups evaluated, and analysis methods were not homogeneous between vaccines, creating uncertainty about some effects and comparisons ( 9 ).
CoronaVac is an inactivated whole-virus vaccine against COVID-19 adjuvanted with aluminum hydroxide created from African green monkey kidney cells (Vero cells) inoculated with SARS-CoV-2 (strain CN02). The Chinese company Sinovac Biotech developed the vaccine, and on June 1, 2021, the World Health Organization (WHO) approved the vaccine for emergency use ( 10 ). Using two 3 μg doses of CoronaVac, the overall efficacy for avoiding symptomatic COVID-19 (before the emergence of concerning variations) has been assessed at 67.7% (95%CI: 35.9 to 83.7%) ( 10 ). Compared to COVID-19 prevention, its impact in preventing hospital stays, ICU admissions, and fatalities has been much stronger. Three-dose regimens have also been shown to raise seroconversion levels of neutralizing antibodies, even against variants like Omicron. Few serious vaccine-related adverse reactions have been reported ( 10 ).
However, given the differences that may exist in the methods used to assess the efficacy, effectiveness, and safety of vaccines against COVID-19, our objective was to explore the published research on COVID-19 vaccines, focusing on CoronaVac, in order to answer the question: How has the efficacy/effectiveness and safety of CoronaVac been assessed in different designs and study phases of the vaccines used to control COVID-19?
A scoping review was carried out under a protocol registered in the Open Science Framework (OSF; osf.io/aeut4), and following the methodology proposed by the Joanna Briggs Institute ( 11 ), which included studies carried out in humans as of 2020, corresponding to systematic reviews, clinical trials, and analytical or descriptive observational studies in which the effectiveness and/or safety of vaccines for COVID19 were evaluated or described. There were no age restrictions on the study participants.
Abstracts from congresses were not evaluated because they had not been subjected to systematic peer evaluation at the time, nor were studies published in languages other than English or Spanish.
2.1 Search methods for study identification
To identify potentially relevant articles for review, the following databases were searched, starting from 2020: MEDLINE, EMBASE, LILACS, Scopus, and Cochrane.
The following valid strategy was used for MEDLINE through PubMed and then adapted to other databases:
(((SARS-CoV-2[MeSH Terms]) OR (COVID-19[MeSH Terms])) OR (Coronavirus[MeSH Terms])) AND ((COVID-19 Vaccines[MeSH Terms]) OR (Coronavirus vaccines[Title/Abstract])).
The full search strategy is presented in the Supplementary material .
2.2 Study selection
The initial screening of the studies was independently performed by two reviewers in pairs (PA-AG and PR-SR). The RIS files of each database were uploaded to Rayyan software ( 12 ). Disagreements were resolved by a third author (JA).
Both reviewers assessed all titles and abstracts and excluded those considered irrelevant for the review, those not meeting the inclusion criteria, or because they were duplicates. Subsequently, 15 reviewers independently (JA, PA, DA, AC, AG, LL, LM, DO, GQ, SR, CR, PR, MS, CT, MA) evaluated the full text of the studies to verify the eligibility criteria. A cross-review was carried out for studies evaluating CoronaVac by four reviewers (PA, AG, PR, and SR).
2.3 Variable
Of the definitively selected studies, the following variables were extracted in a paired form: (i) type of study, (ii) population studied, (iii) intervention (vaccine) evaluated, (iv) control, (v) follow-up time, (vi) efficacy and/or effectiveness outcomes, and (vii) safety outcomes.
2.4 Data synthesis
For each outcome, a description of the results was made following the description in the document and/or Supplementary material of the article.
3.1 Study selection
The search identified 42,813 titles for the initial evaluation, of which 40,372 were excluded after a review of the title, abstract, and possible duplication. A total of 2,441 full texts were reviewed to verify the eligibility criteria, of which 1,685 were included in the synthesis ( Figure 1 ; Supplementary material ).
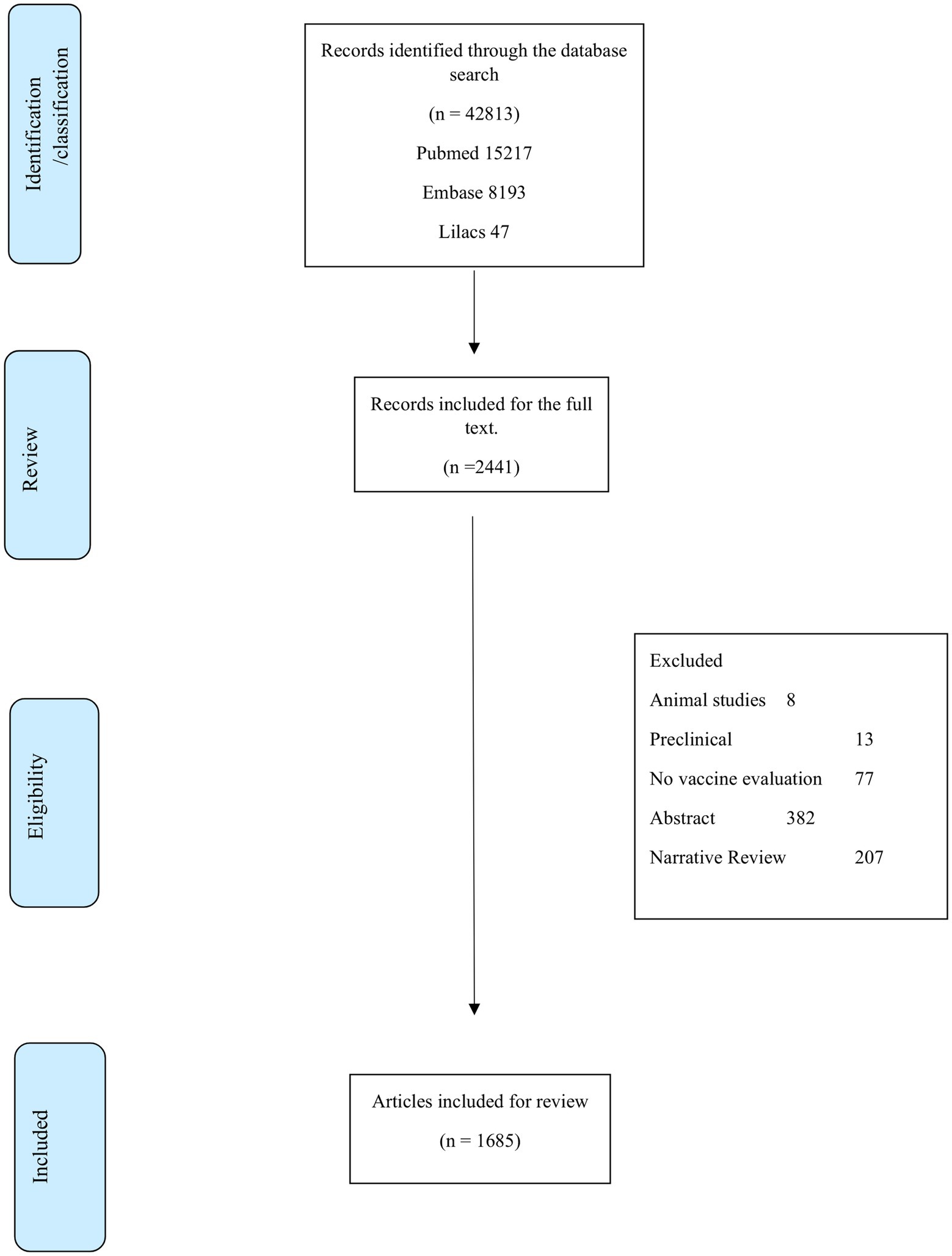
Figure 1 . Flow diagram of the literature review process.
3.2 Synthesis of the results
One hundred vaccines were evaluated through randomized clinical trials (RCT). The other studies corresponded to observational studies, 705 (43.9%) analytical studies, and mainly cohort studies (467; 29.1%). Three hundred and seventy-seven patients (23.5%) were series or case reports.
One hundred twenty-six studies (7.8%) did not specify the vaccine evaluated. Other studies have evaluated one or more specific vaccines. Seven hundred thirty-two studies did not include a vaccine or a control group. Two hundred and thirty-eight evaluated several types of vaccines, and 160 compared a vaccine against a placebo. The number of patients or vaccine doses evaluated in each study went from one (case report) to 306,473,169 doses of applied vaccines ( 13 ).
Regarding the population assessed, 44.4% of the studies evaluated the effects of vaccines on adults. 3.4% in adults and adolescents, 2% in adolescents, 1.2% in immunosuppressed individuals, 1.2% in children, 0.9% in pregnant women, and 0.25% in people living with HIV. The overall monitoring time ranged from hours to 6 months; this difference occurred between studies that evaluated immunological outcomes, which could occur within hours or days, and those that evaluated clinical outcomes.
A total of 15.1% of the studies evaluated the effectiveness or efficacy of vaccines by evaluating their effects on preventing infection, hospitalization, or death from infection. 59.1% of the studies corresponded to the description of safety events. The events were described heterogeneously. In some studies, they are only recorded as “mild adverse events” or “mild systemic events.” Few studies reported specific events such as myocarditis, and hepatic or allergic alterations. Of the studies, 25.8% described immunological outcomes, 368 studies through the measurement of antibodies, and 64 through the effects mediated by cellular immunity.
3.2.1 CoronaVac
The efficacy, effectiveness, and safety of this vaccine have been assessed in 113 studies. Nineteen corresponded to experimental studies, seven of Phase II, five of Phase IV, and four were clinical trials with random assignment, carried out in adults in Chile, Indonesia, and Turkey ( 14 – 17 ), comparing the effect of the vaccine versus placebo. The other studies were observational studies, most of which were case reports, case series, or descriptions of cohorts. Of these, 45.1% were conducted in Asia, 23% in Latin America, and 22.1% in Europe, mainly in Turkey (of 27/29 European studies).
As for the population, 87.6% of the studies were conducted in adults, while the representation of studies in pregnant women, children, immunosuppressed people, or people living with HIV ranged between 0.9 and 3.5% of the studies.
Sixty studies (53.1%) evaluated the effect of CoronaVac in a control group. The others were case reports or descriptions of cohorts without comparison. Of these, 42 (70%) described events in patients who received CoronaVac and another vaccine, without performing an effectiveness or efficacy analysis. Other studies evaluated the efficacy and effectiveness by measuring the effect of preventing hospitalization, death, or COVID. Of the total, 34 studies evaluated CoronaVac (30.1%) and described some immune outcomes.
Although the objective of the review was not to assess the effectiveness of the vaccine, but rather how it has been evaluated, the results of some of the identified studies are shown below in order to present relevant information about the methods used and possible differences between them, which lead to discussing the effect that this can have on the analysis and use of CoronaVac and other vaccines. More details on the results of the identified studies can be found in the Supplementary material section.
3.2.2 Efficacy/effectiveness of CoronaVac
3.2.2.1 prevention of covid-19.
Cheng et al. ( 18 ) evaluated the effectiveness of BNT162B2 and CoronaVac in patients with chronic kidney disease in Hong Kong. 28,374 people were not vaccinated, 27,129 received two doses of BNT162b2, and 47,640 received two doses of CoronaVac in this retrospective cohort analysis. Following inverse probability of treatment weighting with 1% extreme values, a cohort that was well-balanced and had a standardized mean difference of less than 0.1 was generated.
The effectiveness of CoronaVac on Turkish healthcare professionals was assessed by Can et al. ( 19 ). 4,067 medical personnel worked at a University Hospital in Istanbul, where this retrospective cohort study was carried out. In the fully vaccinated group, the follow-up period was defined as beginning 14 days following the second dose. If PCR test findings were positive or the trial came to an end, healthcare personnel were excluded. Healthcare personnel who were not vaccinated were prohibited from participating in any COVID-19 vaccination. The vaccine’s unadjusted and adjusted effectiveness were calculated using the incidence rate ratio and Cox regression. 29% of the healthcare staff had not received any vaccinations, whereas 71% had received all recommended doses.
Jara et al. ( 20 ) conducted an evaluation of a prospective, observational, national-level cohort of individuals (≥ 16 years) associated with the Fondo Nacional de Salud insurance program in Chile. They used individual-level data to assess the efficacy of booster vaccines, namely BNT162B2 (Pfizer-Biontech), AZD1222 (Oxford-AstraZeneca), and CoronaVac (Syovac Biotech), in individuals who had completed a primary immunization schedule with CoronaVac, in comparison to those who had not received any vaccinations. The hazard ratios were estimated using inverse probability-weighted and stratified survival regression models that took into account the time-varying vaccination status and adjusted for pertinent clinical, socioeconomic, and demographic confounders. An estimate was made of the change in risk associated with the primary immunization series and booster shot from being unvaccinated to vaccinated. 11,174,257 persons in total fulfilled the trial’s eligibility conditions; of these, 4,127,546 finished the two doses of the CoronaVac primary immunization regimen and got a booster dose during the study period. 2,019,260 (48.9%) individuals received a BNT162b2 booster, 186,946 (4.5%) received a homologous booster with CoronaVac, and 1,921,340 (46–5%) participants received an AZD1222 booster. The weighted stratified Cox model was utilized to compute the modified vaccination efficacy in preventing COVID-19.
Utilizing hospitalization, vaccination, and National COVID-19 notification data, Cerqueira-Silva et al. ( 21 ) conducted a case–control study in Brazil to evaluate the efficacy of four vaccines (CoronaVac [synovac], ChAdOx1 nCoV-19 [AstraZeneca], Ad26.COV2.S [Janssen], and BNT162b2 [Pfizer-Bionntech]) in individuals with laboratory-confirmed prior SARS-COV-2 infection. The probabilities of test positivity and the likelihood of hospitalization or death from COVID-19 were compared based on vaccination status and the amount of time that had passed from the first or second dose of vaccinations using multivariable conditional logistic regression.
The same authors conducted a similar study in Brazil ( 22 ), using linked national Brazilian databases to conduct a negative-test design study with nearly 14 million participants (~ 16 million tests) to estimate the effectiveness of the CoronaVac vaccine over time and the BNT162B2 booster vaccination against severe COVID-19 outcomes (hospitalization or death) and severe acute respiratory syndrome, as confirmed by RT-PCR (SARS-COV-2).
To evaluate the effectiveness of homologous and heterologous boosters against COVID-19 in the context of OMICRON, Ranzani et al. ( 23 ) conducted a nationwide case–control study (with negative PCR results) to assess homologous and heterologous (BNT162B2) booster doses in adults who received two doses of CoronaVac in Brazil in the OMICRON context.
A case–control research was carried out in Thailand by Sritipsukho et al. ( 24 ) to assess the efficacy of various vaccination regimens in preventing COVID-19 during the time when the delta variant was the predominant causing virus (≥ 95%). By correcting for individual demographic and clinical factors, the efficacy of vaccines was assessed.
3.2.2.2 Prevention of hospitalization and death
Cheng et al. ( 18 ) found that both vaccines reduced hospitalization and death related to COVID-19, which was the opposite of the outcome of preventing COVID-19 infection. The vaccination efficacy for BNT162b2 users was 64% (95% CI: 57–69%) for hospitalization associated to COVID-19 and 86% (95% CI: 80–90%) for COVID-19-related death. Regarding hospitalization and death associated to COVID-19, the vaccine efficacy for CoronaVac was 44% (95% CI: 37–49%) and 70% (95% CI: 64–75%), respectively.
In the Jara et al. ( 20 ) study, the adjusted effectiveness of the vaccine against hospitalization due to COVID-19, ICU admission, and death was 86.3% (83.7–88.5), 92.2% (88.7–94.6), and 86.7% (80.5–91.0) for a CoronaVac homolog booster; 96.1% (95.3–96.9), 96, 2% (94.6–97.3), and 96.8% (93.9–98.3) for a BNT162b2 booster; and 97.7% (97.3–98.0), 98.9% (98.5–99.2), and 98.1% (97.3–98.6) for an AZD1222 booster, respectively.
In Brazil ( 21 ), the effectiveness against hospitalization or death 14 or more days after the completion of the vaccination schedule was 81.3% (75.3–85.8) for CoronaVac, 89.9% (83.5–93.8) for ChAdOx1 nCoV-19, and 57.7% (−2.6–82.5) for Ad26.COV2.S, and 89.7% (54.3–97.7) for BNT162b2.
3.2.2.3 Immunological outcomes
Bueno et al. ( 14 ), conducting a randomized placebo-controlled clinical trial in Chile, assessed the effectiveness of CoronaVac by assigning participants to either a placebo or two doses of CoronaVac spaced 2 weeks apart. Enrollments totaled 434, with 397 individuals in the 18–59 age range and 37 in the 60+ age range. 81 subjects had hemoral assessments. 2 and 4 weeks after the second dosage, respectively, the seroconversion rates for specific anti-S1-receptor binding domain (RBD) immunoglobulin G (IgG) were 82.22 and 84.44% in the 18–59 years age group and 62, 69 and 70.37% in the ≥60 years age group. A notable rise in the amount of neutralizing antibodies in circulation was noted two and 4 weeks following the second dosage. 47 participants had their cells evaluated. After stimulation with Mega Pools of SARS-CoV-2 peptides, a notable increase in T cell responses was seen, as evidenced by the release of interferon-γ (IFN-γ).
According to Zeng et al. ( 25 ) the following were the findings of two single-center, double-blind, randomized, placebo-controlled phase II clinical trials: adults from Jiangsu, China, aged 18 to 59 years were first assigned (1:1) into two vaccination schedule cohorts: one for the days 0 and 14 of vaccination (cohort 1), and another for the days 0 and 28 of vaccination (cohort 2). Each cohort was then randomly assigned (2:2:1) to either a placebo group or a 3 μg or 6 μg dose of CoronaVac. A third dose was given to half of the participants in each cohort 6 months after the second dose, and an additional dose was given to the other half of the individuals 28 days following the second dose, as a result of a protocol revision. In a separate phase II experiment carried out in Hebei, China, individuals who met the eligibility criteria of 60 years or above were randomized to receive three injections of 1.5, 3, or 6 μg of vaccine or a placebo. The first two doses of the vaccine were given 28 days apart, while the second and third doses were given 6 months apart. For the per-protocol population (those who finished their allotted third dose), the primary research outcomes were geometric mean titers (GMTs), geometric mean increments (GMIs), and seropositivity of neutralizing antibodies to SARS-CoV-2. Out of the 600 participants, who were between the ages of 18 and59, 540 (90%) were qualified for a third dose. Of these, 269 (50%) received the third primary dose (cohorts 1a-14d-2 m and 2a-28d-2 m) 2 months after the second dose, and 271 (50%) received a booster dose 8 months later (cohorts 1b-14d-8 m and 2b-28d-8 m). For the 1b-14d-8 m cohort ( n = 53; GMT 3.9 [95% CI 3.1–5.0]) and 2b-28d-8 m cohort ( n = 49; GMT 6.8 [95% CI 5.2–8.8]), neutralizing antibody titers elicited by the first two treatments in the 3 μg group declined after 6 months to close or below the seropositive cut-off point (GMT of 8). The GMTs measured 14 days later increased to 137.9 (95% CI: 99.9–190.4) for the 1b-14d-8 m cohort and to 143.1 (110.8–184.7) 28 days later for the 2b-28d-8 m cohort when a booster dose was administered 8 months following a second dose. After the principal third dosage, GMTs increased somewhat in cohorts 1a-14d-2 m (n = 54) and 2a-28d-2 m ( n = 53). In cohort 1a, GMTs increased from 21.8 (95% CI: 17.3–27.6) on day 28 after the second dose to 45.8 (35.7–58.9) on day 28 after the third dose. Six months following the third dose, GMTs had dropped to almost the positive threshold: in the 1a-14d-2 m group, they were 9.2 (95% CI 7.1–12.0), while in the 2a-28d-2 m cohort, they were 10.0 (7.3–13.7). Similarly, 6 months following the initial two-dose series, neutralizing antibody titers dropped to almost or below the seropositive threshold among people 60 years of age or older who received booster doses (303 [87%] of 350 participants were eligible for a third dosage). Eight months following the second treatment, which markedly raised neutralizing antibody concentrations, a third dose was administered: After the second dose on day 28, GMTs climbed to 42.9 (95% CI: 31.0–59.4), and after the third dose on day 28 ( n = 29), GMTs increased to 158.5 (96.6–259.2).
Chantasrisawad et al. ( 26 ) assessed healthy children aged 5 to 11 who were given two intramuscular doses of either Covilo or CoronaVac and 10 μg of BNT162b2. Neutralizing antibodies against the Omicron version were assessed using a pseudovirus neutralization test (pVNT, ID50) and a surrogate viral neutralization test (sVNT, % inhibition) 14–21 days following the booster. The antibody responses were contrasted with those of a concurrent cohort of kids who got two BNT162b2 doses separated by 3 weeks. A total of 59 children, consisting of 20 CoronaVac recipients and 39 Covilo recipients, were registered between April and May 2022, with a mean age (SD) of 8.5 years (1.7). The primary series’ median interval was 49 days, with an interquartile range of 33–51. Following the booster, the geometric means (MG) of pVNT and sVNT were 499 (95%CI: 399–624) and 72.2% inhibition (95%CI: 67.2–77.6), respectively. From zero to 72 %, the percentage of kids with sVNT against Omicron strain ≥68% inhibition rose. In comparison to the parallel cohort, the geometric mean ratios (GMR) of sVNT and pVNT were 4.3 and 12.2, respectively. In comparison to children who received a booster dosage between 4 and 6 weeks, the GMR of sVNT and pVNT among those who received it at a time interval of more than 6 weeks was 1.2 (95% CI: 1.1–1.3) and 1.8 (95% CI 1.2–2.7).
In Turkey, ( 27 ) et al. assessed the variables influencing the antibody response in 235 adults over 65 years of age following two doses of the inactivated SARS-CoV-2 vaccination (CoronaVac). Four weeks following the first and second vaccination doses, the mean levels of anti-SARS-CoV-2 IgG antibodies were 37.70 ± 57.08 IU/mL and 194.61 ± 174.88 IU/mL, respectively. Additionally, 4 weeks following the first vaccination dose, 134 out of 235 participants (57.02%) had an antibody level of less than 25.6 IU/mL (negative); 4 weeks following the second vaccination dose, this percentage was 11.48% ( n = 27). Eight participants (29.6%) had no comorbidities, while 19 (70.4%) with an antibody level less than 25.6 IU/mL 4 weeks after the first dose of the vaccination had at least one comorbid condition, including diabetes mellitus ( F = 2.352, p = 0.006). Individuals with comorbidities and those 65 years of age or older showed lower antibody response rates.
Demirbakan et al. ( 28 ) examined the presence of immunoglobulin G antibodies in the receptor-binding region of the S1 subunit of the SARS-CoV-2 spike protein in 1072 healthcare workers following immunization in a descriptive observational research. 28 days, 21 days, and 3 months following the first, second, and second dosages, respectively, were the times at which blood samples were taken. Anti-spike antibodies were found in 834/1072 (77.8%) subjects 4 weeks following the initial vaccination dose. Between 18 and 34 years of age, seropositivity was observed to be greater in both men and women (84.6%) compared to 70.6% ( p < 0.001) in the former group. In 1008 of 1,012 (99.6%) cases, anti-spike antibodies were found 21 days after the second dose, and in 803 of 836 (96.1%) cases, anti-spike antibodies were found 3 months later.
3.2.2.4 Safety
According to Bueno et al. ( 14 ) in their placebo-controlled clinical trial, pain at the injection site was the primary adverse reaction in 434 volunteers, and it occurred more frequently in the vaccine arm than in the placebo arm. The majority of the negative effects that were seen were modest and limited. No significant negative events were noted.
The frequency of adverse reactions was reported by Zeng et al. ( 25 ) without providing any additional effect measurements. In every immunization group, all adverse responses that were reported within 28 days after the third dose were classified as either grade 1 or 2. In the 1a-14d-2 m cohort, 150 participants reported three serious adverse events (2%); in the 1b-14d-8 m cohort, 150 participants reported four (3%); in the 2a-28d-2 m and 2b-28d-8 m cohorts, 150 participants reported one (1%); overall 349 people reported 24 (7%) serious adverse events.
Cheng et al. ( 18 ) observed an incidence rate of any adverse events of special interest following the first vaccination dose of 34.28 (95% CI: 29.81–39.23) and 38.39 (95% CI: 34.81–42.23) per 10,000 doses of BNT162b2 and CoronaVac, respectively, in their retrospective cohort of patients with chronic kidney disease. BNT162b2 (incidence rate ratio [95% CI]: first dose: 0.86 [0.69–1.08]; second dose: 0.96 [0.76–1.22]; third dose: 0.60 [0.33–1.10]) and CoronaVac (incidence rate ratio [95% CI]: first dose: 0.76 [0.64–0.91]; second dose: 0.86 [0.71–1.05]; third dose: 0.74 [0.36–1.54]) did not show an increased risk of overall adverse event of special interest when compared to the baseline period.
4 Discussion
The COVID-19 pandemic has affected the world’s population with a high morbidity and mortality rate. Recent reports have described persistent symptoms that extend beyond the initial period of the disease. It has been observed that adverse consequences, in addition to respiratory effects, are produced at different levels: cardiovascular, neurological, or immunological; cutaneous, gastrointestinal, or kidney manifestations, as well as in mental health, both as a result of acute infection and by the so-called post-COVID-19 syndrome ( 29 ). In this context, developing effective and safe vaccines was the determining control measure for pandemic management since, in addition to reducing the transmission of infections and allowing the control of the disease, vaccines had a determining role in reducing severe and fatal complications associated with infection ( 30 ). In addition to the above, the time in which the vaccine candidates were available, where it took less than a year for developers to complete the design, manufacturing, efficacy and safety testing and evaluation and approval for use, is an immeasurable scientific and public health learning, as well as an example of cooperation between healthcare authorities, the scientific community and private sector ( 31 ).
This review presents an analysis of the methods, populations, and scope of the studies that have evaluated the efficacy/effectiveness and safety of the vaccines available for COVID-19, emphasizing CoronaVac. Differences were found in terms of the proportion of populations evaluated, follow-up times, and times of the studies regarding the appearance of variants of concern.
Although some clinical trials with random assignment have been carried out to assess efficiency and safety outcomes with CoronaVac, these have limitations in terms of feasibility, follow-up times, and with this, the possibility of evaluating safety outcomes that occur with low frequencies ( 32 ). In this sense, it is important to carry out observational data analysis. However, not all studies have used homogeneous methods of analysis. Both the prevention of infection, and the prevention of outcomes such as hospitalization or death, have been valued through similar outcomes, but some through multivariate analysis of dependencies, and others through analysis that try to infer causally through different control methods of confounding. Studies have compared the evaluation of the same outcome through different methods, including multivariable logistic regression, propensity matching, propensity adjustment, and propensity-base weighting. However, researchers described that the estimates are very sensitive to the explicit or implicit weighting system in an adjustment technique, so it must be clear for which population a global treatment estimate is most appropriate ( 33 ).
It is important to recognize that there are common challenges in the collection, notification, and use of epidemiological data, such as the exhaustiveness and representativeness of the results and their comparability in time, among others. Therefore, it is necessary to identify the strongest analytical designs (among them the interrupted temporal series and comparative longitudinal studies), accompanied by sensitivity analysis of the results and being explicit, starting from the design, in the type of biases and problems that can be found in the data analysis that is available ( 34 ).
Concerning the evaluation of the immune response to the different types of vaccines, it has been oriented both to the antibody-mediated response and that mediated by cellular immunity. Among the antibody-mediated response, the reference standard has been established with the specific neutralizing antibody response against spike proteins of the virus, and a proxy to this response assessing neutralizing capacity has been measured in other studies by immunoglobulin G (IgG) antibody levels against the SARS-CoV-2 receptor binding domain (RBD) ( 35 ).
In the different studies, the decrease in the response levels to specific neutralizing antibodies was assumed to indicate the vaccine protection level when the levels of specific neutralizing antibodies fell between 4 and 6 months. The statistical methods used for their measurement are not homogeneous among all studies which has been used to recommend the application of boosters with vaccines produced in homologous or heterologous platforms of those received in established vaccination schemes ( 36 , 37 ).
To assess the duration of vaccine protection in the real world, it is also important to consider the difficulties in assessing the cellular memory immune response. The measurement of the CD4+ and CD8+ T lymphocytes response expressed in the production of different activation markers is heterogeneous, depending on antigenic stimuli such as peptides from circulating virus variants, cells from infected individuals, or peptides from different vaccines, in addition to diversity in the host response, which does not allow to have precise indicators to define optimal vaccination schedules ( 38 , 39 ).
In this context, inactivated whole virus vaccines, such as CoronaVac, by preserving epitopes of the virus, could respond in a broader spectrum to the different variants of circulating viruses or to new mutations, which could lead to the optimization of global vaccination schedules ( 10 ).
The main strength of our study lies in its systematic development, which reduces the possibility of biases in study selection. The use of different databases, including Latin American ones, allows for a broader search, although it is acknowledged that due to the magnitude of research on this topic, there may still be unreported or unfound studies, behaving as gray literature. The review results enabled us to achieve our objective, which was to describe how the efficacy/effectiveness of COVID-19 vaccines has been evaluated, with emphasis on CoronaVac. This allowed for the identification of some differences in these methods and some persisting gaps in defining more homogeneous methods for evaluation, regardless of whether these studies had high or low certainty in their evidence, which should be revisited if the objective is to evaluate the effectiveness and/or safety in the population of these interventions. However, the findings presented could be assessed and discussed with broader groups of experts in the field, which would help generate more accurate recommendations regarding their significance and potential implications.
In addition to the mentioned limitations, it is important to acknowledge that this type of review, having less precise question definitions compared to systematic reviews of effectiveness and safety (with their PICO structure), may result in some gaps in the application of search terms that could affect the results. Additionally, the vast amount of information, as was the case in our review, can create difficulties in synthesis and analysis, so it is crucial, as mentioned, to continue the discussion in groups with increasingly greater expertise in the subject ( 40 ). Lastly, while it is tempting to provide quantitative results regarding the synthesis conducted, the most important aspect is to address the original question regarding the gaps in the evaluation of these vaccines.
5 Conclusion
Published information on the evaluation of the efficacy/effectiveness and safety of the different vaccines against COVID-19 is abundant. However, there are differences in terms of vaccine application schedules, population definition, outcomes evaluated, follow-up times, and safety assessment, as well as non-standardization in the reporting of results, which may hinder the generalizability of the findings. It is important to define the relevance of the analysis methods in advance, considering these differences and the heterogeneity that can be produced in the analysis and meta-analysis of this information. It is important to generate meetings and consensus strategies for the methods and reporting of this type of studies, which will allow to reduce the heterogeneity in their presentation and a better understanding of the effect of these vaccines.
Data availability statement
The original contributions presented in the study are included in the article/ Supplementary material , further inquiries can be directed to the corresponding author.
Author contributions
JA-Á: Conceptualization, Methodology, Writing – original draft, Writing – review & editing, Investigation. PA-V: Writing – original draft, Writing – review & editing, Investigation. DA-L: Writing – original draft, Writing – review & editing, Investigation. AC-E: Writing – original draft, Writing – review & editing, Investigation. AG-H: Writing – original draft, Writing – review & editing, Investigation. LL-C: Writing – original draft, Writing – review & editing, Investigation. IM: Writing – original draft, Writing – review & editing, Investigation. DO-L: Writing – original draft, Writing – review & editing, Investigation. GQ: Writing – original draft, Writing – review & editing, Investigation. SR-B: Writing – original draft, Writing – review & editing, Investigation. CR-B: Funding acquisition, Writing – original draft, Writing – review & editing, Investigation. PR: Writing – original draft, Writing – review & editing, Investigation. MS-O: Writing – original draft, Writing – review & editing, Investigation. CT-A: Writing – original draft, Writing – review & editing, Investigation. MA-M: Funding acquisition, Project administration, Writing – original draft, Writing – review & editing, Investigation, Project Administration.
The author(s) declare financial support was received for the research, authorship, and/or publication of this article. CDC-China COVEP Fund Project #211-CO-04 (Submission 03/06/2022). The funders had no role in the design and development of the study, collection, management, analysis, interpretation of the data, writing the report, and the decision to submit the manuscript for publication.
Acknowledgments
We would like to thank Doracelly Hincapié-Palacio and María Teresa Rugeles-López, professors and researchers at the University of Antioquia, for their contributions and suggestions in the discussion of the results.
Conflict of interest
The authors declare that the research was conducted in the absence of any commercial or financial relationships that could be construed as a potential conflict of interest.
Publisher’s note
All claims expressed in this article are solely those of the authors and do not necessarily represent those of their affiliated organizations, or those of the publisher, the editors and the reviewers. Any product that may be evaluated in this article, or claim that may be made by its manufacturer, is not guaranteed or endorsed by the publisher.
Supplementary material
The Supplementary material for this article can be found online at: https://www.frontiersin.org/articles/10.3389/fpubh.2024.1321327/full#supplementary-material
1. Sharma, D, and Shalimar,. COVID-19: finally on wane, with reduced lethality. Comb Chem High Throughput Screen . (2022) 25:768–70. doi: 10.2174/1386207324666210811130046
PubMed Abstract | Crossref Full Text | Google Scholar
2. Hodgson, SH, Mansatta, K, Mallett, G, Harris, V, Emary, KRW, and Pollard, AJ. What defines an efficacious COVID-19 vaccine? A review of the challenges assessing the clinical efficacy of vaccines against SARS-CoV-2. Lancet Infect Dis . (2021) 21:e26–35. doi: 10.1016/S1473-3099(20)30773-8
3. Fang, E, Liu, X, Li, M, Zhang, Z, Song, L, Zhu, B, et al. Advances in COVID-19 mRNA vaccine development. Signal Transduct Target Ther . (2022) 7:94. doi: 10.1038/s41392-022-00950-y
4. Doherty, M, Buchy, P, Standaert, B, Giaquinto, C, and Prado-Cohrs, D. Vaccine impact: benefits for human health. Vaccine . (2016) 34:6707–14. doi: 10.1016/j.vaccine.2016.10.025
Crossref Full Text | Google Scholar
5. Conklin, L, Hviid, A, Orenstein, WA, Pollard, AJ, Wharton, M, and Zuber, P. Vaccine safety issues at the turn of the 21st century. BMJ Glob Health . (2021) 6:898. doi: 10.1136/bmjgh-2020-004898
6. Tregoning, JS, Flight, KE, Higham, SL, Wang, Z, and Pierce, BF. Progress of the COVID-19 vaccine effort: viruses, vaccines and variants versus efficacy, effectiveness and escape. Nat Rev Immunol . (2021) 21:626–36. doi: 10.1038/s41577-021-00592-1
7. Williams, LR, Ferguson, NM, Donnelly, CA, and Grassly, NC. Measuring vaccine efficacy against infection and disease in clinical trials: sources and magnitude of bias in coronavirus disease 2019 (COVID-19) vaccine efficacy estimates. Clin infect dis: official publication of the Infect Dis Society of America agosto de . (2022) 75:e764–73. doi: 10.1093/cid/ciab914
8. Fiolet, T, Kherabi, Y, MacDonald, CJ, Ghosn, J, and Peiffer-Smadja, N. Comparing COVID-19 vaccines for their characteristics, efficacy and effectiveness against SARS-CoV-2 and variants of concern: a narrative review. Clin microbiol infect: official publication of the European Society of Clin Microbiol Infect Dis . (2022) 28:202–21. doi: 10.1016/j.cmi.2021.10.005
9. Graña, C, Ghosn, L, Evrenoglou, T, Jarde, A, Minozzi, S, Bergman, H, et al. Efficacy and safety of COVID-19 vaccines. Cochrane Database Syst Rev . (2022) 2023:477. doi: 10.1002/14651858.CD015477
10. Jin, L, Li, Z, Zhang, X, Li, J, and Zhu, F. CoronaVac: a review of efficacy, safety, and immunogenicity of the inactivated vaccine against SARS-CoV-2. Hum Vaccin Immunother . (2022) 18:2096970. doi: 10.1080/21645515.2022.2096970
11. Peters, MDJ, Godfrey, C, McInerney, P, Munn, Z, Tricco, AC, and Khalil, H. Chapter 11: Scoping Reviews (2020 version). E Aromataris and Z Munn, editors. JBI Manual for Evidence Synthesis. JBI; (2020). Available from https://synthesismanual.jbi.global .
Google Scholar
12. Ouzzani, M, Hammady, H, Fedorowicz, Z, and Elmagarmid, A. Rayyan-a web and mobile app for systematic reviews. Systematic Rev . (2016) 5:210. doi: 10.1186/s13643-016-0384-4
13. Boruah, A, Westenberg, E, Hanif Khan, A, Kee Hoo, F, Guekht, A, Spatola, M, et al. Characterization and analysis of neurologic adverse events associated with COVID-19 vaccination. Neurol Int . (2022) 98:784. doi: 10.1212/WNL.98.18_supplement.3784
14. Bueno, SM, Abarca, K, González, PA, Gálvez, NMS, Soto, JA, Duarte, LF, et al. Safety and immunogenicity of an inactivated severe acute respiratory syndrome coronavirus 2 vaccine in a subgroup of healthy adults in Chile. Clin infect dis: official publication of the Infect Dis Society of America . (2022) 75:e792–804. doi: 10.1093/cid/ciab823
15. Duarte, LF, Gálvez, NMS, Iturriaga, C, Melo-González, F, Soto, JA, Schultz, BM, et al. Immune profile and clinical outcome of breakthrough cases after vaccination with an inactivated SARS-CoV-2 vaccine. Front Immunol . (2021) 12:742914. doi: 10.3389/fimmu.2021.742914
16. Tanriover, MD, Doğanay, HL, Akova, M, Güner, HR, Azap, A, Akhan, S, et al. Efficacy and safety of an inactivated whole-virion SARS-CoV-2 vaccine (CoronaVac): interim results of a double-blind, randomised, placebo-controlled, phase 3 trial in Turkey. Lancet (London, England) . (2021) 398:213–22. doi: 10.1016/S0140-6736(21)01429-X
17. Fadlyana, E, Rusmil, K, Tarigan, R, Rahmadi, AR, Prodjosoewojo, S, Sofiatin, Y, et al. A phase III, observer-blind, randomized, placebo-controlled study of the efficacy, safety, and immunogenicity of SARS-CoV-2 inactivated vaccine in healthy adults aged 18-59 years: an interim analysis in Indonesia. Vaccine . (2021) 39:6520–8. doi: 10.1016/j.vaccine.2021.09.052
18. Cheng, FWT, Fan, M, Wong, CKH, Chui, CSL, Lai, FTT, Li, X, et al. The effectiveness and safety of mRNA (BNT162b2) and inactivated (CoronaVac) COVID-19 vaccines among individuals with chronic kidney diseases. Kidney Int . (2022) 102:922–5. doi: 10.1016/j.kint.2022.07.018
19. Can, G, Acar, HC, Aydin, SN, Balkan, II, Karaali, R, Budak, B, et al. Waning effectiveness of CoronaVac in real life: a retrospective cohort study in health care workers. Vaccine . (2022) 40:2574–9. doi: 10.1016/j.vaccine.2022.03.032
20. Jara, A, Undurraga, EA, Zubizarreta, JR, González, C, Pizarro, A, Acevedo, J, et al. Effectiveness of homologous and heterologous booster doses for an inactivated SARS-CoV-2 vaccine: a large-scale prospective cohort study. Lancet Glob Health . (2022) 10:e798–806. doi: 10.1016/S2214-109X(22)00112-7
21. Cerqueira-Silva, T, Andrews, JR, Boaventura, VS, Ranzani, OT, de Araújo, OV, Paixão, ES, et al. Effectiveness of CoronaVac, ChAdOx1 nCoV-19, BNT162b2, and Ad26.COV2.S among individuals with previous SARS-CoV-2 infection in Brazil: a test-negative, case-control study. The lancet. Infect Dis Ther . (2022) 22:791–801. doi: 10.1016/S1473-3099(22)00140-2
22. Cerqueira-Silva, T, Katikireddi, SV, de Araujo, OV, Flores-Ortiz, R, Júnior, JB, Paixão, ES, et al. Vaccine effectiveness of heterologous CoronaVac plus BNT162b2 in Brazil. Nat Med . (2022) 28:838–43. doi: 10.1038/s41591-022-01701-w
23. Ranzani, OT, Hitchings, MDT, de Melo, RL, de França, GVA, de FR, FC, Lind, ML, et al. Effectiveness of an inactivated Covid-19 vaccine with homologous and heterologous boosters against Omicron in Brazil. Nat Commun . (2022) 13:5536. doi: 10.1038/s41467-022-33169-0
24. Sritipsukho, P, Khawcharoenporn, T, Siribumrungwong, B, Damronglerd, P, Suwantarat, N, Satdhabudha, A, et al. Comparing real-life effectiveness of various COVID-19 vaccine regimens during the delta variant-dominant pandemic: a test-negative case-control study. Emerg Microbes Infect . (2022) 11:585–92. doi: 10.1080/22221751.2022.2037398
25. Zeng, G, Wu, Q, Pan, H, Li, M, Yang, J, Wang, L, et al. Immunogenicity and safety of a third dose of CoronaVac, and immune persistence of a two-dose schedule, in healthy adults: interim results from two single-Centre, double-blind, randomised, placebo-controlled phase 2 clinical trials. Lancet Infect Dis . (2022) 22:483–95. doi: 10.1016/S1473-3099(21)00681-2
26. Chantasrisawad, N, Puthanakit, T, Kornsitthikul, K, Jaru-Ampornpan, P, Tawan, M, Matapituk, P, et al. Immunogenicity to SARS-CoV-2 Omicron variant among school-aged children with 2-dose of inactivated SARS-CoV-2 vaccines followed by BNT162b2 booster. Vaccine: X . (2022) 12:100221. doi: 10.1016/j.jvacx.2022.100221
27. Karamese, M, and Tutuncu, EE. The effectiveness of inactivated SARS-CoV-2 vaccine (CoronaVac) on antibody response in participants aged 65 years and older. J Med Virol . (2022) 94:173–7. doi: 10.1002/jmv.27289
28. Demirbakan, H, Koçer, I, Erdoğan, M, and Bayram, A. Assessing humoral immune response after two doses of an inactivated SARS-CoV-2 vaccine (CoronaVac) in healthcare workers. Public Health . (2022) 205:1–5. doi: 10.1016/j.puhe.2022.01.011
29. Peramo-Álvarez, FP, López-Zúñiga, MÁ, and López-Ruz, MÁ. Medical sequels of COVID-19. Med Clin (Barc) . (2021) 157:388–94. doi: 10.1016/j.medcli.2021.04.023
30. Park, JW, Lagniton, PNP, Liu, Y, and Xu, RH. mRNA vaccines for COVID-19: what, why and how. Int J Biol Sci . (2021) 17:1446–60. doi: 10.7150/ijbs.59233
31. Hodgson, J . The pandemic pipeline. Nat Biotechnol . (2020) 38:523–32. doi: 10.1038/d41587-020-00005-z
32. Gilmartin-Thomas, JF, Liew, D, and Hopper, I. Observational studies and their utility for practice. Aust Prescr . (2018) 41:82–5. doi: 10.18773/austprescr.2018.017
33. Kurth, T, Walker, AM, Glynn, RJ, Chan, KA, Gaziano, JM, Berger, K, et al. Results of multivariable logistic regression, propensity matching, propensity adjustment, and propensity-based weighting under conditions of nonuniform effect. Am J Epidemiol . (2006) 163:262–70. doi: 10.1093/aje/kwj047
34. Stoto, MA, Woolverton, A, Kraemer, J, Barlow, P, and Clarke, M. COVID-19 data are messy: analytic methods for rigorous impact analyses with imperfect data. Glob Health . (2022) 18:2. doi: 10.1186/s12992-021-00795-0
35. Lopera, TJ, Chvatal-Medina, M, Flórez-Álvarez, L, Zapata-Cardona, MI, Taborda, NA, Rugeles, MT, et al. Humoral response to BNT162b2 vaccine against SARS-CoV-2 variants decays after six months. Front Immunol . (2022) 13:879036. doi: 10.3389/fimmu.2022.879036
36. Widge, AT, Rouphael, NG, Jackson, LA, Anderson, EJ, Roberts, PC, Makhene, M, et al. Durability of responses after SARS-CoV-2 mRNA-1273 vaccination. N Engl J Med . (2021) 384:80–2. doi: 10.1056/NEJMc2032195
37. Doria-Rose, N, Suthar, MS, Makowski, M, O’Connell, S, McDermott, AB, Flach, B, et al. Antibody persistence through 6 months after the second dose of mRNA-1273 vaccine for Covid-19. N Engl J Med . (2021) 384:2259–61. doi: 10.1056/NEJMc2103916
38. Primorac, D, Vrdoljak, K, Brlek, P, Pavelić, E, Molnar, V, Matišić, V, et al. Adaptive immune responses and immunity to SARS-CoV-2. Front Immunol . (2022) 13:848582. doi: 10.3389/fimmu.2022.848582
39. Benjamanukul, S, Traiyan, S, Yorsaeng, R, Vichaiwattana, P, Sudhinaraset, N, Wanlapakorn, N, et al. Safety and immunogenicity of inactivated COVID-19 vaccine in health care workers. J Med Virol . (2022) 94:1442–9. doi: 10.1002/jmv.27458
40. Mak, S, and Thomas, A. An introduction to scoping reviews. J Grad Med Educ . (2022) 14:561–4. doi: 10.4300/JGME-D-22-00620.1
Keywords: COVID-19, SARS-CoV-2, vaccines, CoronaVac, review
Citation: Alzate-Ángel JC, Avilés-Vergara PA, Arango-Londoño D, Concha-Eastman A, Garcés-Hurtado A, López-Carvajal L, Minotta IL, Ortega-Lenis D, Quintero G, Reina-Bolaños S, Reina-Bolaños CA, Roa P, Sánchez-Orozco M, Tovar-Acero C and Arbeláez-Montoya MP (2024) How has research on the effectiveness and safety of COVID-19 vaccination been evaluated: a scope review with emphasis on CoronaVac. Front. Public Health . 12:1321327. doi: 10.3389/fpubh.2024.1321327
Received: 13 October 2023; Accepted: 25 March 2024; Published: 10 April 2024.
Reviewed by:
Copyright © 2024 Alzate-Ángel, Avilés-Vergara, Arango-Londoño, Concha-Eastman, Garcés-Hurtado, López-Carvajal, Minotta, Ortega-Lenis, Quintero, Reina-Bolaños, Reina-Bolaños, Roa, Sánchez-Orozco, Tovar-Acero and Arbeláez-Montoya. This is an open-access article distributed under the terms of the Creative Commons Attribution License (CC BY) . The use, distribution or reproduction in other forums is permitted, provided the original author(s) and the copyright owner(s) are credited and that the original publication in this journal is cited, in accordance with accepted academic practice. No use, distribution or reproduction is permitted which does not comply with these terms.
*Correspondence: Juan C. Alzate-Ángel, [email protected]
Disclaimer: All claims expressed in this article are solely those of the authors and do not necessarily represent those of their affiliated organizations, or those of the publisher, the editors and the reviewers. Any product that may be evaluated in this article or claim that may be made by its manufacturer is not guaranteed or endorsed by the publisher.
Featured Clinical Reviews
- Screening for Atrial Fibrillation: US Preventive Services Task Force Recommendation Statement JAMA Recommendation Statement January 25, 2022
- Evaluating the Patient With a Pulmonary Nodule: A Review JAMA Review January 18, 2022
Select Your Interests
Customize your JAMA Network experience by selecting one or more topics from the list below.
- Academic Medicine
- Acid Base, Electrolytes, Fluids
- Allergy and Clinical Immunology
- American Indian or Alaska Natives
- Anesthesiology
- Anticoagulation
- Art and Images in Psychiatry
- Artificial Intelligence
- Assisted Reproduction
- Bleeding and Transfusion
- Caring for the Critically Ill Patient
- Challenges in Clinical Electrocardiography
- Climate and Health
- Climate Change
- Clinical Challenge
- Clinical Decision Support
- Clinical Implications of Basic Neuroscience
- Clinical Pharmacy and Pharmacology
- Complementary and Alternative Medicine
- Consensus Statements
- Coronavirus (COVID-19)
- Critical Care Medicine
- Cultural Competency
- Dental Medicine
- Dermatology
- Diabetes and Endocrinology
- Diagnostic Test Interpretation
- Drug Development
- Electronic Health Records
- Emergency Medicine
- End of Life, Hospice, Palliative Care
- Environmental Health
- Equity, Diversity, and Inclusion
- Facial Plastic Surgery
- Gastroenterology and Hepatology
- Genetics and Genomics
- Genomics and Precision Health
- Global Health
- Guide to Statistics and Methods
- Hair Disorders
- Health Care Delivery Models
- Health Care Economics, Insurance, Payment
- Health Care Quality
- Health Care Reform
- Health Care Safety
- Health Care Workforce
- Health Disparities
- Health Inequities
- Health Policy
- Health Systems Science
- History of Medicine
- Hypertension
- Images in Neurology
- Implementation Science
- Infectious Diseases
- Innovations in Health Care Delivery
- JAMA Infographic
- Law and Medicine
- Leading Change
- Less is More
- LGBTQIA Medicine
- Lifestyle Behaviors
- Medical Coding
- Medical Devices and Equipment
- Medical Education
- Medical Education and Training
- Medical Journals and Publishing
- Mobile Health and Telemedicine
- Narrative Medicine
- Neuroscience and Psychiatry
- Notable Notes
- Nutrition, Obesity, Exercise
- Obstetrics and Gynecology
- Occupational Health
- Ophthalmology
- Orthopedics
- Otolaryngology
- Pain Medicine
- Palliative Care
- Pathology and Laboratory Medicine
- Patient Care
- Patient Information
- Performance Improvement
- Performance Measures
- Perioperative Care and Consultation
- Pharmacoeconomics
- Pharmacoepidemiology
- Pharmacogenetics
- Pharmacy and Clinical Pharmacology
- Physical Medicine and Rehabilitation
- Physical Therapy
- Physician Leadership
- Population Health
- Primary Care
- Professional Well-being
- Professionalism
- Psychiatry and Behavioral Health
- Public Health
- Pulmonary Medicine
- Regulatory Agencies
- Reproductive Health
- Research, Methods, Statistics
- Resuscitation
- Rheumatology
- Risk Management
- Scientific Discovery and the Future of Medicine
- Shared Decision Making and Communication
- Sleep Medicine
- Sports Medicine
- Stem Cell Transplantation
- Substance Use and Addiction Medicine
- Surgical Innovation
- Surgical Pearls
- Teachable Moment
- Technology and Finance
- The Art of JAMA
- The Arts and Medicine
- The Rational Clinical Examination
- Tobacco and e-Cigarettes
- Translational Medicine
- Trauma and Injury
- Treatment Adherence
- Ultrasonography
- Users' Guide to the Medical Literature
- Vaccination
- Venous Thromboembolism
- Veterans Health
- Women's Health
- Workflow and Process
- Wound Care, Infection, Healing
- Download PDF
- Share X Facebook Email LinkedIn
- Permissions
Assessing the Real-World Effectiveness of Immunizations for Respiratory Syncytial Virus
- 1 Surveillance and Prevention Branch, Coronavirus and Other Respiratory Viruses Division, US Centers for Disease Control and Prevention, Atlanta, Georgia
- Medical News & Perspectives “This Is Our COVID”—What Physicians Need to Know About the Pediatric RSV Surge Jennifer Abbasi JAMA
- Medical News in Brief CDC: RSV Vaccine Recommended for Older People Emily Harris JAMA
- Medical News in Brief FDA Approves Maternal RSV Vaccine Emily Harris JAMA
Immunizations for respiratory syncytial virus (RSV) were newly licensed and recommended for 3 populations in the US in 2023, marking a historic turning point in the prevention of RSV illness in both young children and older adults. First discovered in 1956, RSV has long been recognized as a leading cause of acute respiratory illnesses in young children, resulting in an estimated 58 000 to 80 000 hospitalizations in children younger than 5 years of age each year in the US, with infants at greatest risk of hospitalization. 1 Respiratory syncytial virus has also been increasingly recognized as a cause of severe acute respiratory illness in older adults and is estimated to cause 60 000 to 160 000 hospitalizations and 6000 to 10 000 deaths annually among adults aged 65 years or older in the US. 2 Until 2023, only palivizumab (a monoclonal antibody requiring monthly dosing) was available for RSV prevention for eligible infants and young children with certain underlying conditions that increase risk of severe RSV illness.
The US Centers for Disease Control and Prevention now recommends protecting all infants against RSV-associated lower respiratory tract infection during their first RSV season through either the maternal RSV vaccine (Abrysvo [Pfizer]) given to pregnant people or infant receipt of nirsevimab (a long-acting monoclonal antibody). The maternal RSV vaccine is recommended for pregnant persons at 32 to 36 weeks’ gestation using seasonal administration (ie, September through January in most of the continental US) to protect infants after birth through transplacental transfer of maternal antibodies. Nirsevimab is recommended for infants younger than 8 months of age entering their first RSV season and for some children aged 8 to 19 months with certain high-risk conditions entering their second RSV season. For adults aged 60 years or older, 2 RSV vaccines (Arexvy [GSK] and Abrysvo [Pfizer]) are recommended to prevent RSV lower respiratory tract infection.
All 3 newly licensed RSV immunization products were efficacious in preventing severe RSV illness in prelicensure trials. 1 , 3 - 5 Postlicensure studies are needed to corroborate findings from these trials in real-world settings and in populations underrepresented in the prelicensure trials (eg, adults with immunocompromising conditions or frailty and older age groups among adults aged ≥60 years).
Observational studies of real-world immunization effectiveness typically compare the frequency of the illness outcome among persons who were immunized vs those who were not (cohort design) or the frequency of immunization among persons with the illness outcome vs those without the outcome (case-control or test-negative design). In both approaches, uptake of the immunization in the population must be sufficiently high to allow for an effectiveness evaluation. Immunization and outcome status also must be accurately identified. In addition, differences in the characteristics of immunized and unimmunized persons that might be associated with risk of the illness outcome must be addressed in analyses to optimize validity.
The early introduction phase of newly licensed RSV immunizations in the US poses multiple challenges to the assessment of real-world effectiveness and impact. Low immunization uptake among the recommended groups during the 2023-2024 season may hinder assessments of immunization effectiveness using traditional observational study designs. As of February 2024, uptake of the maternal RSV vaccine among eligible pregnant persons was estimated to be 18%, uptake of nirsevimab among infants younger than 8 months of age was 41%, and uptake of RSV vaccines among adults aged 60 years or older was 22%. 6
In addition, there may be differential uptake within groups recommended for immunization, with some subgroups more likely to be immunized than others. For example, among infants and young children, differential uptake of nirsevimab during the 2023-2024 season may have occurred because of early nirsevimab shortages. Similarly, among adults aged 60 years or older, current recommendations use a shared clinical decision-making framework informed by both the patients’ characteristics, values, and preferences and the health care professionals’ clinical discretion. 2 For both older adults aged 60 years or older and pregnant people, vaccination may also occur more frequently in retail pharmacy settings than for other routine vaccines (eg, because of the cost of stocking vaccines and insurance reimbursement mechanisms). Collectively, factors influencing differential uptake within groups recommended for immunization could lead to increased uptake either among those with poorer health and at greater risk of severe RSV illness (some of whom may be at greater risk of suboptimal immune responses to vaccination) or conversely, among healthier individuals who can more easily seek out vaccination. Both phenomena have been observed in studies of influenza vaccine effectiveness and can lead to bias in effectiveness estimates unless patient differences by immunization status and risk of infection are identified, appropriately measured, and addressed in the analyses.
Accurate identification of RSV immunization status may be hampered by patients’ or caregivers’ recall now that up to 3 respiratory virus immunizations (COVID-19 vaccines, influenza vaccines, and RSV immunizations) are available, and timely verification is likely to be challenging because of varying reporting requirements, jurisdictional immunization information systems, and settings for the administration of vaccines and immunizations. To assess effectiveness in young infants, accurate identification of both maternal RSV vaccination and infant immunization (including receipt of both nirsevimab and palivizumab) is important because all 3 immunizations prevent severe RSV illness. Direct comparison of the effectiveness of maternal RSV vaccination vs infant administration of nirsevimab to prevent severe RSV will require adequate use of both strategies in the same source population, standardized outcomes, and rigorous analytic methods to address differences between infants with maternal RSV vaccination exposure vs infant receipt of nirsevimab.
Interpretation of early real-world estimates of RSV immunization effectiveness during the 2023-2024 season may be affected by both patterns of immunization uptake and RSV circulation. Effectiveness estimates for RSV immunization may only be generalizable to groups that had sufficient uptake to study the effectiveness. For example, if nirsevimab was largely given to infants younger than 6 months of age because of updated prioritization guidance during supply shortages, the estimates may not be generalizable to older children. In addition, protection from immunization is expected to wane, especially in the case of passive immunization of infants through maternal vaccination and immunization in infants and young children with long-acting monoclonal antibodies. Immunization effectiveness is likely to appear higher when estimated early in the season with a shorter interval from vaccination or immunization than when estimated for the full season or over multiple seasons. Two early reports 7 , 8 on nirsevimab from Spain and the US suggest high levels of effectiveness against RSV-associated hospitalization early after receipt (with a median interval of 6 weeks from receipt in the US analysis), but emphasize the need for additional estimates of effectiveness for a complete RSV season.
Despite these anticipated challenges, the advent of immunizations for RSV to protect infants and young children and older adults presents unique opportunities to monitor and measure their public health effectiveness and potential shifts in RSV epidemiology. Real-world studies may answer key questions about immunizations for RSV that could not be fully addressed in prelicensure trials, including (1) the durability of protection from RSV immunization; (2) whether effectiveness varies by vaccine product in older adults, strategy (maternal vaccination or infant immunization) in young infants, and risk groups (eg, those with immunocompromising conditions); and (3) whether RSV immunization protects against a broader range of outcomes than studied in the prelicensure trials.
Newly licensed immunizations for RSV have the potential to prevent substantial numbers of hospitalizations and deaths, and real-world evidence of immunization effectiveness may support eventual introduction in low- and middle-income countries where the public health benefit may be greatest. There are rare moments in public health when decades of effort toward prevention of a specific disease culminate in the introduction of new immunization products with the potential to dramatically reduce morbidity and mortality—for RSV, the real-world evidence will determine if that time is now.
Corresponding Author: Fatimah S. Dawood, MD, Coronavirus and Other Respiratory Viruses Division, US Centers for Disease Control and Prevention, 1600 Clifton Rd NE, Atlanta, GA 30307 ( [email protected] ).
Published Online: April 11, 2024. doi:10.1001/jama.2024.5859
Conflict of Interest Disclosures: None reported.
Disclaimer: The findings and conclusions in this report are those of the authors and do not necessarily represent the official position of the US Centers for Disease Control and Prevention.
See More About
Dawood FS , Payne AB , McMorrow ML. Assessing the Real-World Effectiveness of Immunizations for Respiratory Syncytial Virus. JAMA. Published online April 11, 2024. doi:10.1001/jama.2024.5859
Manage citations:
© 2024
Artificial Intelligence Resource Center
Cardiology in JAMA : Read the Latest
Browse and subscribe to JAMA Network podcasts!
Others Also Liked
- Register for email alerts with links to free full-text articles
- Access PDFs of free articles
- Manage your interests
- Save searches and receive search alerts
Serious adverse events of special interest following mRNA COVID-19 vaccination in randomized trials in adults
Affiliations.
- 1 Thibodaux Regional Health System, Thibodaux, LA, USA. Electronic address: [email protected].
- 2 Unit of Innovation and Organization, Navarre Health Service, Spain. Electronic address: [email protected].
- 3 Institute of Evidence-Based Healthcare, Bond University, Gold Coast, QLD, Australia. Electronic address: [email protected].
- 4 Fielding School of Public Health and College of Letters and Science, University of California, Los Angeles, CA, USA. Electronic address: [email protected].
- 5 Geffen School of Medicine, University of California, Los Angeles, CA, USA. Electronic address: [email protected].
- 6 Clinical Excellence Research Center, School of Medicine, Stanford University, CA, USA. Electronic address: [email protected].
- 7 School of Pharmacy, University of Maryland, Baltimore, MD, USA. Electronic address: [email protected].
- PMID: 36055877
- PMCID: PMC9428332
- DOI: 10.1016/j.vaccine.2022.08.036
Introduction: In 2020, prior to COVID-19 vaccine rollout, the Brighton Collaboration created a priority list, endorsed by the World Health Organization, of potential adverse events relevant to COVID-19 vaccines. We adapted the Brighton Collaboration list to evaluate serious adverse events of special interest observed in mRNA COVID-19 vaccine trials.
Methods: Secondary analysis of serious adverse events reported in the placebo-controlled, phase III randomized clinical trials of Pfizer and Moderna mRNA COVID-19 vaccines in adults ( NCT04368728 and NCT04470427 ), focusing analysis on Brighton Collaboration adverse events of special interest.
Results: Pfizer and Moderna mRNA COVID-19 vaccines were associated with an excess risk of serious adverse events of special interest of 10.1 and 15.1 per 10,000 vaccinated over placebo baselines of 17.6 and 42.2 (95 % CI -0.4 to 20.6 and -3.6 to 33.8), respectively. Combined, the mRNA vaccines were associated with an excess risk of serious adverse events of special interest of 12.5 per 10,000 vaccinated (95 % CI 2.1 to 22.9); risk ratio 1.43 (95 % CI 1.07 to 1.92). The Pfizer trial exhibited a 36 % higher risk of serious adverse events in the vaccine group; risk difference 18.0 per 10,000 vaccinated (95 % CI 1.2 to 34.9); risk ratio 1.36 (95 % CI 1.02 to 1.83). The Moderna trial exhibited a 6 % higher risk of serious adverse events in the vaccine group: risk difference 7.1 per 10,000 (95 % CI -23.2 to 37.4); risk ratio 1.06 (95 % CI 0.84 to 1.33). Combined, there was a 16 % higher risk of serious adverse events in mRNA vaccine recipients: risk difference 13.2 (95 % CI -3.2 to 29.6); risk ratio 1.16 (95 % CI 0.97 to 1.39).
Discussion: The excess risk of serious adverse events found in our study points to the need for formal harm-benefit analyses, particularly those that are stratified according to risk of serious COVID-19 outcomes. These analyses will require public release of participant level datasets.
Keywords: Adverse events of special interest; Brighton Collaboration; COVID-19; COVID-19 vaccines; Coalition for Epidemic Preparedness Innovations; Moderna COVID-19 vaccine mRNA-1273; NCT04368728 ; NCT04470427 ; Pfizer-BioNTech COVID-19 vaccine BNT162b2; SARS-CoV-2; Safety Platform for Emergency vACcines; Serious adverse events; Vaccines; mRNA vaccines.
Copyright © 2022 Elsevier Ltd. All rights reserved.
Publication types
- Clinical Trial, Phase III
- Randomized Controlled Trial
- COVID-19 Vaccines / adverse effects
- COVID-19* / prevention & control
- RNA, Messenger
- Randomized Controlled Trials as Topic
- Vaccination / adverse effects
- Vaccines, Synthetic
- mRNA Vaccines
- COVID-19 Vaccines
Associated data
- ClinicalTrials.gov/NCT04368728
- ClinicalTrials.gov/NCT04470427
- Open access
- Published: 06 June 2023
Uptake and determinants of childhood vaccination status among children aged 0–12 months in three West African countries
- Amadou Barrow 1 , 2 ,
- Ayobami Oyekunle Afape 4 ,
- Dalanda Cham 1 , 3 &
- Precious Chidozie Azubuike 5
BMC Public Health volume 23 , Article number: 1093 ( 2023 ) Cite this article
3493 Accesses
2 Citations
1 Altmetric
Metrics details
Vaccination has long been recognized as one of the most effective ways to reduce child mortality. It has played a significant role, particularly for children, and is considered a major achievement and relevant in preventing childhood diseases worldwide. This study looks at the uptake and determinants of childhood vaccination status among children under the age of one year, for Gambia, Sierra Leon, and Liberia.
Data from 2019 to 20 Demographic and Health Survey (DHS) data from Gambia, Sierra Leone, and Liberia were pooled for the analysis used in this study. Data were obtained from a total weighted sample of 5,368 children aged 0–12 months through a stratified two-stage cluster sampling approach. A multivariable logistic regression model was used to assess the predictors of childhood vaccination uptake at 95% confidence interval (CIs) with computed adjusted odds ratios (aORs).
The weighted sample pooled prevalence of full vaccination among children under 12 months of age was 15.1% for males and 15.0% for females. After controlling for confounders in the regression model, factors that were found to be associated with vaccination status include children whose mothers attended postnatal care (PNC) visits had higher odds of being fully vaccinated (aOR = 1.23, 95% CI = 1.03–1.46), while children whose fathers had primary education (aOR = 0.67, 95% CI = 0.48–0.96), children whose households never watched TV (aOR = 0.68, 95% CI = 0.56–0.82) and children whose mothers attended 1–3 antenatal care (ANC) visits (aOR = 0.59, 95% CI = 0.45–0.79) had lower odds of being fully vaccinated.
Childhood vaccination uptake was low among children under 12 months of age in these countries. Hence, there is a need to promote the uptake of vaccination across these three West African countries especially among rural dwellers.
Peer Review reports
Introduction
Vaccination has long been recognized as one of the most effective ways to reduce child mortality [ 1 ]. It has played a significant role, particularly for children, and is considered a major achievement and relevant in preventing childhood diseases worldwide. In practice, the terms “vaccination” and “immunization” are interchangeable [ 2 ]. Improving access to immunization is critical to meeting the Sustainable Development Goals (SDGs). Childhood vaccination is highly effective in preventing vaccine-preventable diseases [ 1 ]. The discovery and introduction of the smallpox vaccine have greatly reduced disease prevalence worldwide, with 29 vaccine-preventable illnesses recommended by the World Health Organization (WHO) [ 3 ]. Despite the numerous ways to reduce the number of children dying from vaccine-preventable diseases, immunizations have improved immunity in 9 out of every 10 children [ 2 ]. Vaccines are very effective when major risk factors like malnutrition, air pollution and diarrheal diseases are eliminated, as well as treatment accessibility is improved.
Poor adherence to vaccination still remains a challenge in many regions across the world [ 4 ]. Globally, it is estimated that about 22.4 million children under one year of age were not vaccinated with the third dose of pentavalent vaccine (Diphtheria Pertussis-Tetanus-Hepatitis B-Haemophilus type b influenza vaccine (DPT-HB-Hib) [ 3 ]. One out of five African children is estimated to not receive all the necessary and basic vaccinations. Consequently, over 30 million African children under five still suffer from vaccine-preventable diseases (VPDs) every year. Approximately 58% of all deaths due to VPDs occur in children over half a million a year [ 5 ]. Several factors contribute to vaccines’ low coverage as highlighted in the literature. Households, lack of communication, and knowledge about immunization have been reported to be major contributors [ 6 ]. One of the major contributors to vaccine utilization in Africa highlighted is economic conditions, which affect the ability to afford and keep immunization record documents in good condition [ 7 ]. A study published in the literature looked into cultural perspectives as a contributor to low vaccination coverage. It was discovered to be linked with people’s misinformed perceptions of vaccines due to their cultural background [ 8 ]. In Pakistan, is linked to the father’s job as manual labour [ 9 ]. In addition, mothers’ perception of the severity of vaccine-preventable disease, as well as mothers’ beliefs do not influence the uptake as reported in a study conducted in Tanzania [ 10 ].
Sub-Saharan Africa is still dealing with a variety of program and policy challenges related to childhood immunization [ 11 ]. Numerous studies have been conducted to investigate factors associated with childhood immunization in Sub-Saharan Africa, some of which indicate parental attitudes, a lack of knowledge among health workers, and barriers that can be overcome by improving outreach services [ 12 ]. Furthermore, a study conducted in Burkina Faso discovered that education, both at the individual and community levels, is no longer associated with complete vaccination of children [ 13 ]. This demonstrates that there are differences in factors within the African region, such as the need for uptake. It has been observed that many countries in the sub-Saharan African region have proven to have a high vaccination coverage, but due to various limitations have not achieved this upscaled coverage, necessitating the need to study underlying factors [ 11 ].
Several West African immunization programs have made significant progress in increasing vaccination coverage, which is expected to have a significant impact on the reduction of vaccine-preventable diseases [ 1 ]. The Gambia, Sierra Leon, and Liberia for example, have shown that the immunization strategies are effective when there are good political and cultural will; however, vaccination programs should be constantly monitored and evaluated by the Ministries of Health. To raise societal awareness and vaccine acceptance, strong community-based health education efforts are desperately needed as part of initiatives to increase vaccine service utilization for these high-risk classes. This study examines the uptake and determinants of childhood vaccination status among children under the age of one, using the available DHS data for Gambia, Sierra Leon, and Liberia.
Data source
The 2019-20 Demographic and Health Survey (DHS) data from Gambia, Sierra Leone, and Liberia were pooled for the analysis. In the three countries, the DHS used a stratified two-stage cluster sampling approach to create a population-based sample. Following the probability proportional to the size of the Enumerated Areas (EAs), 281 (The Gambia), 325 (Liberia), and 578 (Sierra Leone) clusters/EAs were selected in the first stage of the survey. The second stage involved a methodical selection of 25 (The Gambia), 30 (Liberia), 24 (Sierra Leone) households from each cluster/EA and only 8,362 (The Gambia), 5, 704 (Liberia), 9,889 (Sierra Leone) women of reproductive age with children less than 60 months were interviewed successfully. In DHS, specific questions were asked to women about children’s health. Questions related to immunization coverage are of particular interest. We pooled the DHS survey data of the three countries, and a total weighted sample of 5,368 children aged 0–12 months was included in the study. In the three countries, through the USAID-funded MEASURE DHS programme, ICF International provided technical and financial assistance to the Ministry of Health in collaboration with the Bureau of Statistics of each country that implemented the survey.
Variable selection and measurement
Outcome variables . The study outcome variable was childhood vaccination status among children aged 0–12 months. This was classified into two categories: “Fully vaccinated” a child under 23 months who received WHO recommended vaccination against tuberculosis (also known as BCG), three doses of DPT-HepB-Hib (Penta), three doses of polio vaccines, and one dose of vaccination against measles; “Partially vaccinated” who missed at least one of any of the doses of the routine vaccines before turning 1 year or within 12–23 months old [ 14 , 15 , 16 , 17 , 18 ].
Explanatory variables . Thirteen independent variables were utilized in the study based on a thorough literature review and datasets availability [ 14 , 15 , 16 ]; the variables are listed in Table 1 .
The WHO framework on epidemiology of the unimmunized child [ 19 , 20 ] describes the different factors affecting child’s immunization into four main categories: health care immunization system, communication and information, family characteristics, and parental attitudes and knowledge [ 21 ]. In our study, the immunization system category included the distance to health facility, and the need to take transportation. The communication and information category included: use of mass media according to the levels of access and source (radio, TV and newspapers), family characteristics included the followings variables: mother’s and father’s education level, mother’s age at childbirth, marital status, household level of poverty assessed by the wealth quintile, ethnic group, religion, child gender, birth order, urban/rural residence (urban/rural), and region of residence. Variables on familiarity and use of other health care services such as antenatal care during pregnancy, postnatal care, and the relative distance to the closest health center represented the parental attitudes/knowledge. Finally, we included the gender relationship such as the involvement of women in household decision making.
Statistical analysis
Stata survey (‘svy’) module was used to adjust for stratification, clustering and sampling weights provided in the dataset. The data was weighted (v005/1,000,000) throughout the analysis to ensure DHS sample representativeness and to obtain reliable estimates and standard errors before data analysis. Descriptive statistics were used to describe the level of immunization coverage by socio-demographic characteristics, the distributions were expressed as frequencies and percentages. Bivariate and multivariable logistic regression analyses were conducted to identify the determinants of full immunization. Bivariate analyses were used to examine the crude association between each independent variable and full vaccination. Multivariable logistic regression was used to examine the adjusted association between each independent variable and full childhood vaccination. Binary logistic regression was chosen because our dependent variable was dichotomous (i.e., 0 - partially vaccinated and 1- fully vaccinated). Variables in bivariate analysis with p-values less than 0.05 were entered into the multivariable analysis. Adjusted odds ratio (aOR) and 95% confidence Interval (CI) were used to assess the strength of associations between the outcome and the independent variables. The threshold for statistical significance was set at p < 0.05. We used the Bayesian Deviance Information Criterion (BIC) to assess the goodness of fit of the model. Variance Inflation Factor (VIF) was applied to test for multicollinearity. All the study data were analyzed using Stata version 17 and IBM SPSS version 25.
Ethical approval
The datasets used in this research were population-based datasets that are freely available in the public domain. For reasons of confidentiality, specific characteristics that could be used to identify participants in the study were excluded. As a secondary study, MEASURE DHS/ICF International granted the authors permission to use the datasets.
Socio-demographic characteristics of infants’ mothers in Gambia, Liberia and Sierra Leone
Based on the result from the pooled data as shown in Table 2 , a total of 5,368 children aged 0 and 12 months were pooled across the three West African countries for the study. Child characteristics showed that about half (52.1%) of the children in the study sample were males and 47.9% were females. The family/parental characteristics showed that the mean age of child’s mother and father (± SD) was 27 years (± 6.8) and 38 years (± 11.1) respectively, almost half of the mothers (44.7%) and 49.5% of the fathers had no formal education. 86.3% of the mothers had more than 4 ANC visit, 42.8% never attended PNC, 79.0% delivered in public hospitals and 53.8% of the fathers made decision on vaccination. Household characteristics showed that among the respondents, 50.3% lived in rural area, 22.9% were among the poorest wealth quintile and 61.5% practiced Islam. Communication and information characteristics showed that 92.9% never read newspaper, 38.7% sometimes listened to radio, and 42.9% sometimes watched TV.
Table 3 : Bivariate analysis of factors associated with childhood vaccination among infant aged 0–12 months .
Similar proportions of male (15.1%) and female children (15.0%) reportedly had full vaccination. Children whose mother were 25–34 years (16.3%) and 35 & above (14.4%) had higher proportion of full vaccination compared to those less than 24 years (13.9%) as shown in Table 3 . Children whose mother had no education (14.4%) had lower full vaccination status compared to those with secondary (16.1%) and tertiary (16.1%) education. The proportion of full vaccination was higher among children who lived in urban area (15.8%) compared to those who resided in the rural area (14.3%). Higher proportion of full vaccination was recorded among children whose mothers were married (15.4%) compared to those single (13.8%) or widowed/divorced (12.3%). Higher proportion of full vaccination was recorded among those who sometimes watched TV (18.1%) or listened to radio (15.8%) or read newspaper (16.2%). The higher proportion of full vaccination was recorded among children whose mother had no ANC visit (19.6%) and had PNC visit (16.5%). Lower proportion of full vaccination status was recorded among those whose mothers delivered at home (12.8%) and had big problem about the distance to the health facility (12.8%). Bivariate analysis indicated that father’s education, religion, watching TV and listening to radio, ANC visit, PNC visit, distance to health facility were significant risk factors associated with full vaccination among children 0–12 months in three West African countries.
Determinants of childhood vaccination among infant aged 0–12 months in the three west african countries
Table 4 shows the binary logistic regression results on the factors associated with childhood full vaccination in three West African countries. In the adjusted model, children whose mother attended PNC visit had higher odds (aOR = 1.23, 95% CI = 1.03–1.46) of being fully vaccinated compared to those who had no visit. Religion was significantly associated with full vaccination. Children whose father had primary education had lower odds (aOR = 0.67, 95% CI = 0.48–0.96) of being fully vaccinated compared to those who had no education. Children whose household never watched TV had lower odds (aOR = 0.68, 95% CI = 0.56–0.82) of full vaccination compared to those who watched sometimes. Finally, also children whose mother attended one to three ANC visit had lower odds (aOR = 0.59, 95% CI = 0.45–0.79) of being fully vaccinated compared to those who had more than four visit.
Immunization has been shown to have a significant impact on vaccine preventable diseases. Despite these success and progress, vaccinations uptake is currently faced with several challenges, such as unequal access, lack of resources and vaccine hesitancy [ 5 , 22 , 23 , 24 , 25 ]. The study found that religion, fathers’ education, and PNC visits are all factors that hinder vaccination uptake in children under one year of age. These findings are similar to other studies that reported culture, religion and community belief systems that were reported as potential barriers or deterrents to vaccine uptake in Africa [ 22 , 26 , 27 , 28 ]. The study also revealed that children’s vaccination uptake is influenced by the socioeconomic and residential status of their caregivers. Families with higher incomes who live in cities have a better chance of getting their children vaccinated than low-income families who live in rural areas. Similar studies supported the critical influence of family support systems toward improving vaccine coverages among infants in Sub-Saharan African countries [ 22 , 23 , 27 ].
The study found that mothers who visited postnatal care had a higher chance of having their children vaccinated as compared to mothers who had no previous PNC visits. This is consistent with a study conducted in Benin and the findings revealed that children whose mothers had no antenatal care visits had a lower likelihood of receiving full immunization than those whose mothers had 1–3 visits. This demonstrates that contact with health care workers and a better understanding of vaccinations are factors that influence a child being vaccinated. However, institutional mistrust especially across local health authorities within the bigger picture of health systems related factors toward child vaccination uptake has created barriers and unequal access to immunization services and coverages in Africa [ 22 , 24 ]. Parents can play an important role in ensuring their children are vaccinated, as evidence on the many benefits of vaccinations abound. However, there are other issues relating with the parents that affect vaccine uptake. The study’s findings also revealed that religion played a significant role in children’s vaccination uptake. This is consistent with the study conducted in Nigeria, which shows that religious belief was associated with the non-uptake of vaccination for children by their caregivers[ 29 ]. However, this was not the case in Burkina Faso as religion was contributary to partial immunization [ 30 ]. Religious beliefs shape how people see the world and determine how they live in many African settings [ 22 , 23 ]. However, we can argue that individual levels of belief differ across regions and within countries, and thus how people live and what is accepted may influence vaccination uptake [ 23 ].
Furthermore, education was identified as a determinant of childhood vaccination uptake in the three countries. The study revealed that fathers with at least a primary level of education have a higher likelihood of their child being vaccinated than those with no formal education. This corroborate findings from a study conducted by Anu Rammohan et al., which revealed that in the six countries studied, having a father with a secondary (high school) education or higher was statistically significant and positively correlated with the likelihood of a child receiving measles vaccination, even if the mother is illiterate[ 31 ]. The findings were consistent with the study conducted in southeast Ethiopia that revealed that paternal education was also found to be statistically associated with child immunization status in a cross-sectional study of 591 children aged 12–23 months. In the study, children of fathers with secondary or higher education levels were three times more likely to be fully vaccinated than children whose fathers had no formal education [ 32 ]. This could be attributed to cultural influences in various African settings. In many instances, the decision of the family solely depends on the man, reflecting the patriarchal nature of African societies [ 25 , 33 ]. This is a system where the educational levels of men as heads of households/families continuous to be influenced by culture and tradition.
Awareness of the importance of vaccination, as well as dangers of not being vaccinated demonstrates evidence that may explain why parents who have access to information and watch TV are more likely to have their children fully vaccinated. This is because, they may obtain information from a health facility, the media, or other sources and is consistent with the Ghanaian study [ 34 ]. The study findings demonstrate how vaccination knowledge and understanding can significantly influence vaccination uptake among children in African settings. In Mali, studies show that a lack of information and inconvenience led to only partial immunization of children [ 35 ]. This means that healthcare workers play a significant role, as do awareness campaigns that could be launched to educate the public and encourage positive behaviour. Using pooled data, from the Gambia, it was found that male children were fully vaccinated more than female children. This significantly differ from results in Liberia and Sierra Leone, where no significant differences were recorded in child sex and vaccination status. Furthermore, the pooled data showed that, the age of parents was significantly higher in Libera when compared to Gambia and Sierra Leone.
The governments of these nations need to strengthen their commitment towards WHO’s 2030 immunization agenda, which envisions a world where everyone, everywhere, at any age, has access to vaccines for good health and well-being [ 36 ]. Thus, a value-based Global Immunization Strategy is clearly needed, with the aim of putting citizens/populace at the center [ 37 , 38 ]. There is also a need to set priorities for action to be implemented in the three African countries studied, for designing of an all-inclusive, integrated, and culturally adaptive new immunization strategies to be implemented. Some unpublished grey literature from Gambia reported that community-based immunization defaulter tracing strategies that are tailored in context-specific local settings have the potential to improve clinic attendance for childhood vaccination. These could also have the potential to influence political decisions that prioritizes immunization programs and strategies for local populace.
Study limitations
Since this survey was cross-sectional, causal relationships between variables of interest could not be definitively determined. There might be some level of recall bias in the study and non-response could also influence the accuracy of the data.
The prevalence of childhood vaccination uptake was low among children under 12 months of age and associated factors were number of mothers’ PNC visits, fathers’ educational level, access to watch TV as well as mothers’ number of ANC visits. There is a need to promote the uptake of childhood vaccination uptake across these three countries, especially among rural dwellers. Government should design robust, community-based social and behavioral change communication strategies and programs with strong elements of awareness raising at household and community levels.
Data Availability
Data for this study were sourced from Demographic Health Survey (DHS)and available here: https://www.dhsprogram.com/data/available-datasets.cfm .
World Health Organization P, Immunization. https://www.who.int/news-room/facts-in-pictures/detail/immunization . Accessed 6 Apr 2022.
World Health Organization. Vaccine-preventable diseases and vaccines. 2016. https://www.who.int/ith/ITH-Chapter6.pdf . Accessed 4 Apr 2022.
World Health Organization, Vaccines. and immunization. 2022. https://www.who.int/health-topics/vaccines-and-immunization#tab=tab_1 . Accessed 4 Apr 2022.
Armstrong EP. Economic benefits and costs Associated with Target Vaccinations. J Manag Care Pharm. 2007;13(7 Supp B):12–5.
Google Scholar
World Health Organization. Business case for WHO immunization activities on the African continent 2018–2030. WHO | Regional Office for Africa. 2023. https://www.afro.who.int/publications/business-case-who-immunization-activities-african-continent-2018-2030 . Accessed 6 May 2023.
Sanou A, Simboro S, Kouyaté B, Dugas M, Graham J, Bibeau G. Assessment of factors associated with complete immunization coverage in children aged 12–23 months: a cross-sectional study in Nouna district, Burkina Faso. BMC Int Health Hum Rights. 2009;9:10.
Article Google Scholar
Makenga G, Bonoli S, Montomoli E, Carrier T, Auerbach J. Vaccine production in Africa: a Feasible Business Model for Capacity Building and sustainable new vaccine introduction. Front Public Health. 2019;7:56.
Article PubMed PubMed Central Google Scholar
Luo C, Chen A, Cui B, Liao W. Exploring public perceptions of the COVID-19 vaccine online from a cultural perspective: semantic network analysis of two social media platforms in the United States and China. Telemat Inf. 2021;65:101712.
Bugvi AS, Rahat R, Zakar R, Zakar MZ, Fischer F, Nasrullah M, et al. Factors associated with non-utilization of child immunization in Pakistan: evidence from the demographic and Health Survey 2006-07. BMC Public Health. 2014;14:232.
Chambongo PE, Nguku P, Wasswa P, Semali I. Community vaccine perceptions and its role on vaccination uptake among children aged 12–23 months in the Ileje District, Tanzania: a cross section study. Pan Afr Med J. 2016;23.
Bangura JB, Xiao S, Qiu D, Ouyang F, Chen L. Barriers to childhood immunization in sub-saharan Africa: a systematic review. BMC Public Health. 2020;20:1108.
Rainey JJ, Watkins M, Ryman TK, Sandhu P, Bo A, Banerjee K. Reasons related to non-vaccination and under-vaccination of children in low and middle income countries: findings from a systematic review of the published literature, 1999–2009. Vaccine. 2011;29:8215–21.
Article PubMed Google Scholar
Sia D, Fournier P, Kobiané J-F, Sondo BK. Rates of coverage and determinants of complete vaccination of children in rural areas of Burkina Faso (1998–2003). BMC Public Health. 2009;9:416.
Touray E, Barrow A, Kinteh B, Badjie M, Nget M, Touray J, et al. Childhood vaccination uptake and associated factors among children 12–23 months in rural settings of the Gambia: a community-based cross-sectional study. BMC Public Health. 2021;21:1740.
Tamirat KS, Sisay MM. Full immunization coverage and its associated factors among children aged 12–23 months in Ethiopia: further analysis from the 2016 Ethiopia demographic and health survey. BMC Public Health. 2019;19:1019.
Adedokun ST, Uthman OA, Adekanmbi VT, Wiysonge CS. Incomplete childhood immunization in Nigeria: a multilevel analysis of individual and contextual factors. BMC Public Health. 2017;17:236.
World Health Organization. Summary of WHO Position Papers - Recommendations for Routine Immunization. 2023. https://cdn.who.int/media/docs/default-source/immunization/immunization_schedules/table_1_feb_2023_english.pdf?sfvrsn=c7de0e97_11&download=true . Accessed 6 May 2023.
World Health Organization. Summary of WHO Position Papers - Recommendations for Routine Immunization for Children. 2023. https://cdn.who.int/media/docs/default-source/immunization/immunization_schedules/table_2_feb_2023_english.pdf?sfvrsn=3e27ab48_11&download=true . Accessed 6 May 2023.
World Health Organization. Epidemiology of the Unimmunized child: findings from the Grey Literature. WHO; 2009.
Rutstein SO, Rojas G. Guide to DHS Statistics: Demographic and Health Surveys Methodology. 2006.
Mbengue MAS, Sarr M, Faye A, Badiane O, Camara FBN, Mboup S, et al. Determinants of complete immunization among senegalese children aged 12–23 months: evidence from the demographic and health survey. BMC Public Health. 2017;17:630.
Adamu AA, Essoh T-A, Adeyanju GC, Jalo RI, Saleh Y, Aplogan A, et al. Drivers of hesitancy towards recommended childhood vaccines in african settings: a scoping review of literature from Kenya, Malawi and Ethiopia. Expert Rev Vaccines. 2021;20:611–21.
Article CAS PubMed Google Scholar
Gebeyehu NA, Asmare Adela G, Dagnaw Tegegne K, Birhan Assfaw B. Vaccination dropout among children in Sub-Saharan Africa: systematic review and meta-analysis. Hum Vaccines Immunother. 2022;18:2145821.
Stoop N, Hirvonen K, Maystadt J-F. Institutional mistrust and child vaccination coverage in Africa. BMJ Glob Health. 2021;6:e004595.
Sikweyiya Y, Addo-Lartey AA, Alangea DO, Dako-Gyeke P, Chirwa ED, Coker-Appiah D, et al. Patriarchy and gender-inequitable attitudes as drivers of intimate partner violence against women in the central region of Ghana. BMC Public Health. 2020;20:682.
Cooper S, Schmidt B-M, Sambala EZ, Swartz A, Colvin CJ, Leon N et al. Factors that influence parents’ and informal caregivers’ views and practices regarding routine childhood vaccination: a qualitative evidence synthesis. Cochrane Database Syst Rev. 2021;2021.
Goldstein S, MacDonald NE, Guirguis S. Health communication and vaccine hesitancy. Vaccine. 2015;33:4212–4.
Madhi SA, Rees H. Special focus on challenges and opportunities for the development and use of vaccines in Africa. Hum Vaccines Immunother. 2018;14:2335–9.
Adebayo B. Vaccination Resistance, Religion and Attitudes to Science in Nigeria.:285.
Antai D. Faith and child survival: the role of religion in childhood immunization in Nigeria. J Biosoc Sci. 2009;41:57–76.
Rammohan A, Awofeso N, Fernandez RC. Paternal education status significantly influences infants’ measles vaccination uptake, independent of maternal education status. BMC Public Health. 2012;12:336.
Legesse E, Dechasa W. An assessment of child immunization coverage and its determinants in Sinana District, Southeast Ethiopia. BMC Pediatr. 2015;15:31.
Gruenbaum E, Earp BD, Shweder RA. Reconsidering the role of patriarchy in upholding female genital modifications: analysis of contemporary and pre-industrial societies. Int J Impot Res. 2023;35:202–11.
Adokiya MN, Baguune B, Ndago JA. Evaluation of immunization coverage and its associated factors among children 12–23 months of age in Techiman Municipality, Ghana, 2016. Arch Public Health. 2017;75:28.
Koumaré AK, Traore D, Haidara F, Sissoko F, Traoré I, Dramé S, et al. Evaluation of immunization coverage within the expanded program on immunization in Kita Circle, Mali: a cross-sectional survey. BMC Int Health Hum Rights. 2009;9:13.
World Health Organization. Immunization Agenda 2023: A global strategy to leave no one behind. 2022. https://cdn.who.int/media/docs/default-source/immunization/strategy/ia2030/ia2030-draft-4-wha_b8850379-1fce-4847-bfd1-5d2c9d9e32f 8.pdf?sfvrsn=5389656e_69&download=true. Accessed 6 May 2023.
Calabro’ GE, Carini E, Tognetto A, Giacchetta I, Bonanno E, Mariani M, et al. The value(s) of vaccination: building the scientific evidence according to a value-based Healthcare Approach. Front Public Health. 2022;10:786662.
De Waure C, Calabrò GE, Ricciardi W. Recommendations to drive a value-based decision-making on vaccination. Expert Rev Vaccines. 2022;21:289–96.
Download references
Acknowledgements
The authors thank the MEASURE DHS project for their support and for free access to the original data.
The authors have no support or funding to report.
Author information
Authors and affiliations.
Department of Public & Environmental Health, School of Medicine & Allied Health Sciences, University of The Gambia, Kanifing, The Gambia
Amadou Barrow & Dalanda Cham
Department of Epidemiology, University of Florida, Gainesville, United States of America
Amadou Barrow
Epidemiology & Disease Control Unit, Ministry of Health, Kotu, The Gambia
Dalanda Cham
Kaduna State Primary Health Care and Development Board, Kaduna State, Nigeria
Ayobami Oyekunle Afape
Department of Public Health, University of Calabar, Calabar, Nigeria
Precious Chidozie Azubuike
You can also search for this author in PubMed Google Scholar
Contributions
AB, AA, DC & AP conceptualized the study and prepared the study design, reviewed literature, analysed data and wrote the results. All authors critically reviewed the manuscript for its intellectual content. AB had final responsibility to submit for publication.
Corresponding author
Correspondence to Amadou Barrow .
Ethics declarations
Ethics approval and consent to participate.
Ethics approval for this study was not required since the data is secondary and is available in the public domain. More details regarding DHS data and ethical standards are available at: http://goo.gl/ny8T6X .
Consent for publication
No consent to publish was needed for this study as we did not use any details, images or videos related to individual participants. In addition, data used is available in the public domain.
Competing interests
The authors declare no competing interests.
Additional information
Publisher’s note.
Springer Nature remains neutral with regard to jurisdictional claims in published maps and institutional affiliations.
Rights and permissions
Open Access This article is licensed under a Creative Commons Attribution 4.0 International License, which permits use, sharing, adaptation, distribution and reproduction in any medium or format, as long as you give appropriate credit to the original author(s) and the source, provide a link to the Creative Commons licence, and indicate if changes were made. The images or other third party material in this article are included in the article’s Creative Commons licence, unless indicated otherwise in a credit line to the material. If material is not included in the article’s Creative Commons licence and your intended use is not permitted by statutory regulation or exceeds the permitted use, you will need to obtain permission directly from the copyright holder. To view a copy of this licence, visit http://creativecommons.org/licenses/by/4.0/ . The Creative Commons Public Domain Dedication waiver ( http://creativecommons.org/publicdomain/zero/1.0/ ) applies to the data made available in this article, unless otherwise stated in a credit line to the data.
Reprints and permissions
About this article
Cite this article.
Barrow, A., Afape, A., Cham, D. et al. Uptake and determinants of childhood vaccination status among children aged 0–12 months in three West African countries. BMC Public Health 23 , 1093 (2023). https://doi.org/10.1186/s12889-023-15863-w
Download citation
Received : 27 October 2022
Accepted : 10 May 2023
Published : 06 June 2023
DOI : https://doi.org/10.1186/s12889-023-15863-w
Share this article
Anyone you share the following link with will be able to read this content:
Sorry, a shareable link is not currently available for this article.
Provided by the Springer Nature SharedIt content-sharing initiative
- Children under 12 months
- Vaccination
- Determinants
BMC Public Health
ISSN: 1471-2458
- Submission enquiries: [email protected]
- General enquiries: [email protected]
- Open access
- Published: 08 March 2022
WHO-led consensus statement on vaccine delivery costing: process, methods, and findings
- Ann Levin 1 ,
- Laura Boonstoppel 2 na1 ,
- Logan Brenzel 3 na1 ,
- Ulla Griffiths 4 na1 ,
- Raymond Hutubessy 5 na1 ,
- Mark Jit 6 na1 ,
- Vittal Mogasale 7 na1 ,
- Sarah Pallas 8 na1 ,
- Stephen Resch 9 na1 ,
- Christian Suharlim 9 na1 &
- Karene Hoi Ting Yeung 5 na1
BMC Medicine volume 20 , Article number: 88 ( 2022 ) Cite this article
2550 Accesses
10 Citations
2 Altmetric
Metrics details
Differences in definitions and methodological approaches have hindered comparison and synthesis of economic evaluation results across multiple health domains, including immunization. At the request of the World Health Organization’s (WHO) Immunization and Vaccines-related Implementation Research Advisory Committee (IVIR-AC), WHO convened an ad hoc Vaccine Delivery Costing Working Group, comprising experts from eight organizations working in immunization costing, to address a lack of standardization and gaps in definitions and methodological guidance. The aim of the Working Group was to develop a consensus statement harmonizing terminology and principles and to formulate recommendations for vaccine delivery costing for decision making. This paper discusses the process, findings of the review, and recommendations in the Consensus Statement.
The Working Group conducted several interviews, teleconferences, and one in-person meeting to identify groups working in vaccine delivery costing as well as existing guidance documents and costing tools, focusing on those for low- and middle-income country settings. They then reviewed the costing aims, perspectives, terms, methods, and principles in these documents. Consensus statement principles were drafted to align with the Global Health Cost Consortium costing guide as an agreed normative reference, and consensus definitions were drafted to reflect the predominant view across the documents reviewed.
The Working Group identified four major workstreams on vaccine delivery costing as well as nine guidance documents and eleven costing tools for immunization costing. They found that some terms and principles were commonly defined while others were specific to individual workstreams. Based on these findings and extensive consultation, recommendations to harmonize differences in terminology and principles were made.
Conclusions
Use of standardized principles and definitions outlined in the Consensus Statement within the immunization delivery costing community of practice can facilitate interpretation of economic evidence by global, regional, and national decision makers. Improving methodological alignment and clarity in program costing of health services such as immunization is important to support evidence-based policies and optimal resource allocation. On the other hand, this review and Consensus Statement development process revealed the limitations of our ability to harmonize given that study designs will vary depending upon the policy question that is being addressed and the country context.
Peer Review reports
Immunization has been shown to provide a high return on investment across low- and middle-income countries [ 1 ]. Nevertheless, disparities in immunization access persist within and between countries. With the launch of the new Immunization Agenda 2030 [ 2 ], many low- and middle-income countries (LICs and MICs) are considering introducing new vaccines or vaccine-related technologies, life-course immunization programs, and improving the effectiveness and efficiency of their immunization programs. To determine the feasibility of doing so, estimation of vaccine procurement and delivery costs is of considerable interest to policymakers, program managers, researchers, and other stakeholders concerned with improving immunization programs. In particular, results from delivery cost studies can help countries in decision-making and planning on introducing new infant and life-course vaccines and technologies, preparation of budgets and financing for rollout of vaccines, and evaluation of alternative service delivery approaches.
Recent reviews of immunization delivery cost literature identified a lack of standardization in methods and reporting, making cross-study comparison and synthesis difficult [ 3 ]. These discrepancies limit the interpretability and utility of immunization cost study evidence for immunization program decision-making. In light of these challenges, the World Health Organization (WHO) Immunization and Vaccines-related Implementation Research Advisory Committee (IVIR-AC) recommended at their March 2018 meeting that the WHO Guidance on Vaccine Delivery Costing be updated [ 4 ]. An ad hoc Working Group comprising vaccine delivery costing experts Footnote 1 was therefore convened by the WHO secretariat to review guidance documents and tools on vaccine delivery costing, focused on low- and middle-income country settings. This initial review found that several groups were already developing methodological guidance to address the disparate definitions and approaches in the field, which partly address the original IVIR-AC’s request. In March 2019, IVIR-AC modified its request to instead review guidance documents and costing tools, assess their similarities and differences, and identify gaps in guidance [ 5 ]. In addition, the Working Group recommended that a Consensus Statement be developed to harmonize the differences in costing terminology and principles for groups working in vaccine delivery costing.
For the purpose of this paper, vaccine delivery costing is defined as “costs associated with delivering immunizations to target populations, exclusive of vaccine procurement costs” [ 6 ].
This paper describes the history and process involved to develop the Consensus Statement on Vaccine Delivery Costing, the methods used and the findings of the review of guidance documents and costing tools, and terms and principles as well as recommendations agreed upon by the Working Group.

Process of developing the consensus statement
The consultation process of coming to agreement on a Consensus Statement included setting up a time-limited Working Group of staff of organizations working in vaccine delivery costing (who are also the authors of this paper), conducting a review of guidance documents, costing tools, and other documents and the costing terms, methods, and principles used in these, agreeing upon the costing terminologies and principles, making recommendations to harmonize their differences, writing the text of the Consensus Statement and Annexes (Additional file 1 ). Figure 1 shows a timeline of the meetings and activities that led to the development of the Consensus Statement.
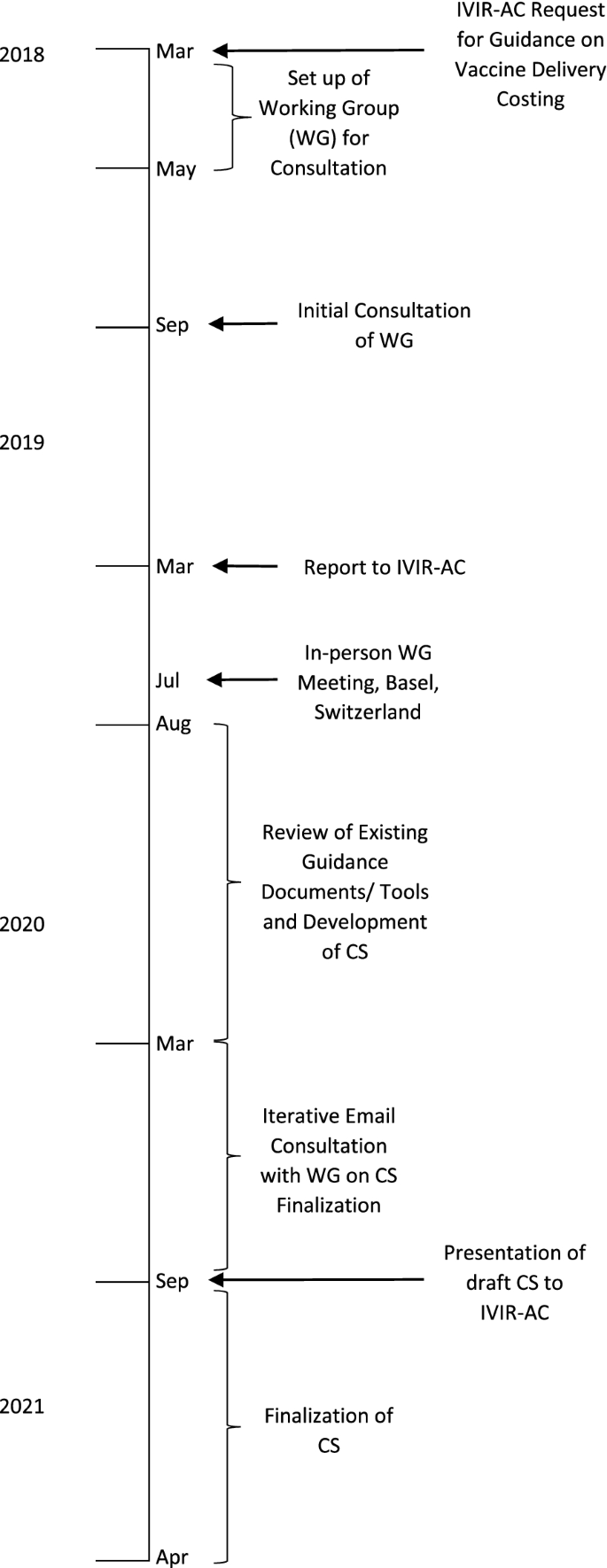
Timeline of the consultation process to develop a Consensus Statement (CS) on vaccine delivery costs
In March 2018, the IVIR-AC initiated the process and requested that WHO update its guidance for conducting vaccine delivery costing in LICs and MICs so that methods used in costing tools and guidance documents could be standardized among WHO and other organizations.
As a follow-up, the WHO secretariat set up a Working Group of Experts comprising staff of organizations conducting research and policy advice on vaccine delivery costing in LICs and MICs to ensure that no parallel efforts were taking place. The initial Working Group comprised of technical experts from the Bill & Melinda Gates Foundation (BMGF), International Vaccine Institute (IVI), Levin & Morgan LLC, UNICEF, and WHO, and two members of IVIR-AC. The group noted that there are several ongoing workstreams conducting cost studies and developing guidance documents and/or costing tools, with different purposes and approaches to costing. In addition, some of these workstreams had developed guidance documents specific to their approach, which were already in the public domain. Thus, a review of these would be required to determine if an additional vaccine delivery costing guidance would be necessary. The Working Group also suggested that a presentation be made at the IVIR-AC meeting in March 2019 to present the findings on the different workstreams to determine the next steps.
In March 2019, the WHO team and the BMGF-funded ThinkWell project (Immunization Costing Action Network [ICAN]) presented to IVIR-AC on findings from the discussion with the Working Group [ 2 ]. Footnote 2 IVIR-AC recommended that an in-person workshop meeting be held with other groups working on vaccine delivery costing so that a consensus could be reached on the best way to standardize costing terms and principles.
In July 2019, WHO and the BMGF convened a meeting with eleven experts from different organizations and institutions in immunization economics during an International Health Economics Association (iHEA) meeting in Basel, Switzerland. The Working Group was expanded to include technical experts from other organizations involved in vaccine delivery costing such as Harvard (Expanded Programme on Immunization Costing [EPIC] studies) and the United States Centers for Disease Control and Prevention (CDC). Based on the subject matter knowledge and professional experience of the Working Group members, the different purposes of the workstreams were discussed and a matrix of costing tools listing out the characteristics of each was created. The Working Group agreed that it would be useful to develop a Consensus Statement that presents the different purposes of each workstream, a review of existing vaccine delivery costing guidance documents and tools, and agreed-upon costing terms and principles.
As a follow-up from the meeting in Basel, from August 2019 to March 2020, an analysis of guidance documents and tools was conducted for each of the four workstreams identified by the Working Group. The Group identified similarities and differences in costing methods, terms and principles among the approaches and in guidance documents, and gaps where further guidance was needed.
Based on these findings, the WHO team developed a proposed draft Consensus Statement report with recommendations for costing terms and principles that could be adhered to for future vaccine delivery costing work and accompanying annexes that summarized the findings from the review on costing terms, costing principles, and methods for vaccine delivery costing. After extensive consultation within the Working Group and several rounds of written revisions to reach consensus on the statement, the findings and recommendations were presented to IVIR-AC in September 2020. The IVIR-AC commended the process to create the Consensus Statement (Additional file 1 ) [ 7 ].
Review of vaccine delivery costing guidance documents and tools
The first step was to conduct a landscape analysis of the organizations involved in vaccine delivery costing and their workstreams, and the available guidance documents and tools on vaccine delivery costs. This landscape analysis was conducted through discussions between the Working Group members during teleconferences and an in-person meeting as well as internet searches of websites of organizations working in the field (e.g., ICAN, Immunization Economics) between August 2019 and March 2020. It was not a systematic literature review and did not aim to include general health service costing tools and guidance documents beyond those with known use for costing immunization in LICs and MICs. However, the analysis built on the recent systematic review and reporting guidance for immunization costing studies conducted by some working group member organizations [ 3 ].
The second step was to compare the characteristics of the guidance documents and tools for immunization costing identified in terms of (1) how costing terms were defined in the guidance documents and costing tools; (2) whether data collection, sampling, and analysis were described in the guidance documents; and (3) whether costing principles were specified in guidance documents.
To review the costing terms in the guidance documents, the definitions were extracted from the source documents and entered into a table so that similarities and differences could be compared qualitatively and recommendations could be made for harmonized definitions for key terms. The costing principles and the guidance text, including on data collection, sampling, and analysis, were also compared and entered into a table by workstreams to assess the similarities, differences, and gaps. To do so, the costing principles in the guidance documents were compared to the ones in the checklist in the Global Health Cost Consortium (GHCC) [ 8 ] that has become a normative reference standard for global health costing work. These principles are similar to those found in the CHEERS checklist [ 9 ]. Recommendations were then made for harmonized principles in the Consensus Statement.
Existing immunization delivery costing workstreams
The Working Group identified four major current workstreams on vaccine delivery costing in LICs and MICs. These include the following: (1) retrospective routine immunization (multiple vaccines) cross-sectional costing, (2) retrospective single-vaccine costing, (3) new vaccine introduction cost projection, and (4) national immunization program cost projection (Fig. 2 ). Although the workstreams had involvement from particular organizations at the time of the review, they are defined by their different objectives and corresponding methodologies and constitute a typology of immunization delivery costing work to which other organizations and practitioners beyond those listed contribute.
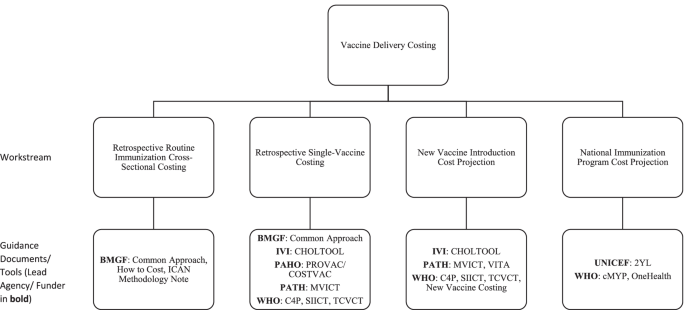
Major current workstreams in vaccine delivery costing identified by the Working Group. Note: 2YL, 2nd Year of Life; BMGF, Bill & Melinda Gates Foundation; C4P, Cervical Cancer Prevention and Control Costing; CDC, United States Centers for Disease Control and Prevention; CHOLTOOL, Oral Cholera Vaccine Costing Tool; cMYP, comprehensive multi-year plan; EPIC, Expanded Programme on Immunization Costing; ICAN, Immunization Costing Action Network; IVI, International Vaccine Institute; MVICT, Malaria Vaccine Immunization Costing Tool; SIICT, Seasonal Influenza Immunization Costing Tool; TCVCT, Typhoid Conjugate Vaccine Costing Tool; VTIA, Vaccine Technology Costs and Health Impact Assessment Tool; WHO, World Health Organization
The first workstream is focused on estimating retrospective (i.e., already incurred) routine immunization cross-sectional costs of service delivery units at a single point in time for multiple vaccines delivered through the routine immunization program. These analyses focus on estimating routine immunization costs incurred at the facility, district, and higher administrative levels in the health system. Such analyses typically estimate unit costs (cost per dose, cost per person, or cost per fully immunized person [FIP]). Some examples of this work include the EPIC studies [ 10 ] and other work by institutes, such as the Harvard T.H. Chan School of Public Health, Wits University, Curatio Foundation, PAHO, ThinkWell, UNICEF, Johns Hopkins University, and PATH. The objectives of research within this workstream are to develop benchmarks for costs to be used in future studies, to analyze variation in unit costs, and to compare the findings with data from other costing studies [ 4 ].
The second workstream is to estimate retrospective costs for a specific vaccine or campaign, typically using incremental costing. That is, it usually aims to measure the value of additional resources employed to introduce a new vaccine or conduct a vaccination campaign. This is often done through data collection at a single point in time (post-campaign or post-introduction) with reference to documents and recall by key informants to estimate which resource use was specifically incremental. Examples of such studies include those conducted by groups such as EPIC, ThinkWell, CDC, and IVI. This workstream includes retrospective cost studies of vaccine implementation using vaccine-specific costing tools (e.g., Cervical Cancer Prevention and Control Costing [C4P], Oral Cholera Vaccine Costing Tool [CHOLTOOL], Malaria Vaccine Immunization Costing Tool [MVICT], Seasonal Influenza Immunization Costing Tool [SIICT], and Typhoid Conjugate Vaccine Costing Tool [TCVCT]). These studies yield results that will assist countries with comparing budgeted amounts to actual implementation resource use, budgeting for future immunization activities, and conducting cost-effectiveness analyses that compare the incremental resource use for a specific vaccine introduction or campaign with its incremental health impacts.
The third workstream is focused on estimating new vaccine introduction costs through projection of the value of resources or ingredients (e.g., time, equipment, training, and vaccines) needed for vaccine introduction, typically using incremental costing for a specific period. Data for these analyses are obtained through interviews with program managers and facility visits to obtain current information on personnel time, supplies, equipment, and other resources as well as retrospective cost data from other vaccine introduction. These analyses are often conducted using costing tools, including some of the same tools used for retrospective single-vaccine costing (e.g., C4P, CHOLTOOL, MVICT, SIICT, and TCVCT). These studies produce cost estimates that will assist countries with planning and decision-making on new vaccines during the introduction period.
The fourth workstream is projection of immunization program costs. Some costing tools used to produce these estimates include the comprehensive multi-year plan (cMYP), 2nd Year of Life (2YL), and OneHealth tool where the activities of a national program and related cost is entered for a baseline year and then the future years are projected. These analyses are an integral part of strategic planning for budgeting and resource mobilization over a specific period of time such as 5 years. Whereas work under the first three workstreams may produce estimates of financial, economic, or undepreciated financial costs, projections under the fourth workstream are intended to estimate undepreciated financial costs (i.e., undiscounted monetary outlays).
In practice, projects may combine elements of multiple workstreams (e.g., retrospective single vaccine costing in one country may be used to help inform estimates of new vaccine introduction costs for a different vaccine or delivery strategy).
Existing guidance documents on vaccine delivery costing
Table 1 shows the nine guidance documents on vaccine delivery costing identified by the Working Group. Some of these provide guidance for more than one type of costing. Three are for estimation of retrospective routine immunization cross-sectional costs, five are for estimation of retrospective single-vaccine costs, five are for projection of new vaccine introduction costs, and one for projection of immunization program costs. The list of costing tools for vaccine delivery identified by the Working Group is shown in Additional file 1 : Table A2b.
Table 2 shows a comparison of costing term definitions among the various guidance documents. It shows that among the different guidance documents, definitions are generally similar but have differences in wording, e.g., vaccine delivery cost, economic cost, start-up/ introduction cost, and prospective cost. Also, some terms (retrospective costing, cost projections, bottom-up and top-down costing) are only defined in the Global Health Costing Consortium reference case. Note that some guidance documents have been grouped together since they were developed by the same teams; i.e., (i) EPIC documents and (ii) WHO vaccine-specific costing tool user manuals.
Figure 3 shows the percentage of guidance documents with definitions of individual costing terms. As can be seen, most documents had definitions of financial cost, economic cost, capital cost, recurrent cost, incremental cost, and vaccine delivery cost, and about half of these defined start-up/introduction cost. Fewer than half of the guidance documents had definitions of perspective, micro-costing (ingredients costing), full costing, retrospective costing, or cost projection.
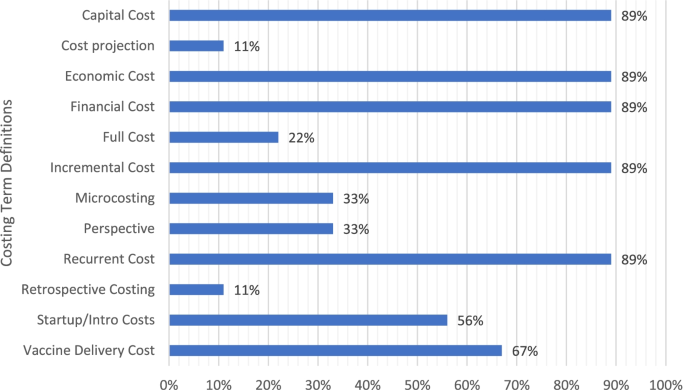
Percentage of guidance documents with definitions of costing terms ( N = 9)
Several gaps were noted from the review. Most guidance documents did not go into detail about some methodological decision points in costing, such as how the choice of perspective will affect which costs are included as financial costs, which may limit the comparability of such costs across studies. For example, if a payer or provider perspective is used, the organizations included in the study definition as “payers” or “providers” will determine whose monetary outlays are considered as financial costs. If a donor (e.g., Gavi) provides funding to a UNICEF country office for social mobilization for a new vaccine introduction, expenditures of those funds will be included as financial costs only if the study perspective is defined as including UNICEF (e.g., a provider perspective defined as all partners “providing” the new vaccine introduction activities, or a health sector perspective including all health sector partners); however, if the study is conducted from a perspective that does not include UNICEF (e.g., a provider perspective defined as only the government “providing” the new vaccine introduction activities, or a government perspective), these resources from UNICEF would not be counted as financial costs but only as economic costs as an in-kind contribution from UNICEF.
Also, most guidance documents did not address whether to include economic costs of existing capital such as equipment or building space, or how to make or assess assumptions for slackness (i.e., available unused capacity) of existing capital goods. Also, vaccine delivery costing definitions differ on whether actual vaccine product costs should be included or not. If not, which specific aspects of the vaccine product costs should be excluded (e.g., vaccine only, diluent, syringes, safety boxes, freight, and insurance). For financial costs, the guidance review suggested whether to include existing personnel costs will depend on whether the costing is incremental or full.
Figure 4 shows the percentage of documents that recommended key costing principles (details in Additional file 1 : Table A3). As can be seen, most guidance documents recommended principles on stating objectives, defining units, describing time horizon, methods and data sources, and annualizing capital costs, while less than half recommend specifying the perspective, scope, sampling, data collection timing, discount rates, shadow prices, exploring variation, analyzing uncertainty, and methods of communicating results.
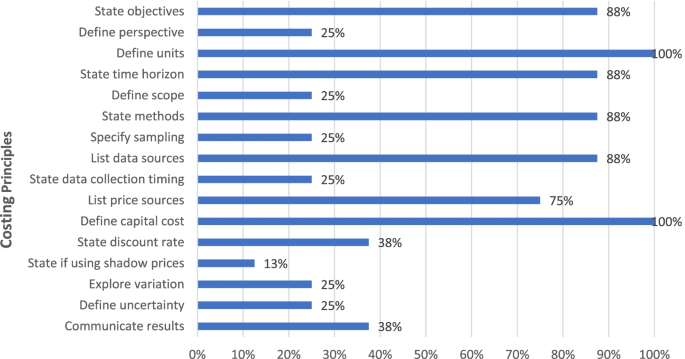
Percentage of costing principles recommended by guidance documents ( N = 9)
In Table 3 , the recommendations of guidance documents on data collection and analysis are disaggregated by workstream. While guidance is given on some aspects in all documents, in other cases, no guidance is provided. Specifically, guidance is given on data collection for all of the workstreams with the exception of projection of new vaccine introduction costs.
Recommended costing terminology and principles
After reviewing the definitions of costing terms, the following definitions of costing terms are recommended:
Vaccine delivery costs
Costs associated with delivering immunization programs to target populations, exclusive of vaccine costs.
Vaccine cost
At a minimum includes the cost of the vaccine and diluent (if applicable); the analysis should include accounting for wastage rates; the analyst should specify whether this also includes injection supplies (syringes), international shipment, insurance, and customs/duties.
Financial cost
Monetary outlays, with straight-line depreciation for capital goods; does not include opportunity costs for use of resources or donated goods and services from sources other than the payer(s) defined in the analysis. Definition is dependent on perspective since monetary outlays are specific to the payer(s) defined in the analysis.
Economic cost
The value of all resources utilized, regardless of the source of financing. Includes opportunity costs for use of existing resources and any donated goods or services from any source. Capital costs are annualized and discounted.
Undepreciated financial cost
Financial costs without depreciation of capital costs (note: such costs have been termed “initial investment” in some costing tools and referred to as fiscal costs in previous analyses.)
Recurrent cost
Value of resources that last less than one year. Start-up activity costs may include recurrent costs.
Capital cost
Value of resources lasting more than one year such as equipment, buildings, and trainings. Start-up activity costs may include capital costs.
Incremental cost
Cost of adding a new service/intervention or a package of services/interventions over and above an existing program; inclusion of existing resources will depend on assumptions made about excess capacity (i.e., whether resources are underemployed; if there are no slack resources (e.g., all personnel time is fully allocated before the addition of the new service/intervention), then their use for the new service or intervention incurs an opportunity cost that should be included—either by measurement or assumption).
Baseline cost as well as the additional/incremental cost of the new intervention, including vaccine cost.
Cost projection
Estimation of future costs of both recurrent and capital inputs.
Prospective data collection
Direct observation of resource use during intervention implementation; i.e., data are collected concurrently with intervention implementation.
Retrospective data collection
Data collection after resource use is completed.
Start-up cost
Cost of initial one-time programmatic activities. Examples may include initial micro-planning, initial training activities, and initial sensitization/social mobilization/information, education, and communication (IEC); does not include routine or repeated programmatic activities such as refresher training or annual microplanning. Start-up activities may include both recurrent and capital costs; they are defined by the non-repeating nature of the activity, not the type of input.
Micro-costing
Focuses on granular accounting of input prices and quantities; disaggregates costs of particular output into specific goods and services consumed.
Bottom-up costing
Measures input quantities at the client (e.g., per vaccination administered) or activity level.
Top-down costing
Divides overall program cost or expenditures, often including those at administrative levels above service delivery level, by number of outputs to calculate unit cost.
Perspective
The point of view considered for costs (and benefits, if included) in a costing study, by whom the costs were incurred. Payers are the disbursing agents for a good or service, and may differ from the original source of funding. A provider perspective includes costs incurred by health service providers (can be limited to the government), a payer perspective includes costs to the payer(s), such as government or an external partner, while the societal perspective includes all costs incurred by providers as well as clients.
Shared cost
Shared resources that are not used only for immunization, but also for other productive activities.
The recommended costing principles include the following.
Definitions of terms used in studies of vaccine delivery costing should conform closely to the recommended definitions in this Consensus Statement.
The study scope in terms of its purpose, audience, target population, time horizon, and service/output should be clearly stated. It should also state whether data collection will be prospective or retrospective, and whether the analysis will be retrospective or a cost projection.
The perspective of the cost estimation should be stated and justified.
Types of costs to be generated should be clearly defined in terms of start-up/introduction or non-start-up/introduction (sometimes called operating costs), recurrent and capital, undepreciated financial, financial or economic, and incremental or full. Capital costs should be appropriately annualized and depreciated for financial and economic costs and the discount rate justified.
The scope of the inputs to be estimated should be defined, justified, and if needed referenced. For example, do the costs include national and sub-national costs or only facility-level service delivery costs? Are non-immunization costs included?
The “units” in the unit costs for strategies, services, and interventions should be defined, e.g., cost per dose administered.
If incremental costing is conducted, any assumptions made regarding existing health system capacity should be described (see GHCC reference case, pg. 64).
The selection of the data sources, including any adjustments to price data (e.g., inflation or currency conversion) should be described and referenced.
The methods for estimating the quantity of inputs should be described—whether top-down or bottom-up, methods of allocation, use of shadow prices and the opportunity cost of time, and methods for excluding research and evaluation costs.
Costs should be mapped and reported as either inputs or activities:
Resource inputs include, for example, personnel time, vaccines, injection and safety supplies, vehicles, fuel, per diem and travel allowances, cold chain equipment, stationery, laboratory equipment, and buildings;
Program activities include, for example, vaccine procurement, service delivery, training, micro-planning, social mobilization, and advocacy and communication, monitoring and evaluation, surveillance, adverse event following immunization monitoring, and supervision.
Some boundaries around costs included in the analysis may be employed to keep the costing scope feasible and will depend on the purpose of the costing study, with the rationale for any exclusions provided; use discretion about including one-time costs that are unique or unlikely to be replicated or transferable across settings (for example, new vaccine launches with the President). Clarify definition and threshold for including or excluding small costs that have expected small contribution (e.g., <$25) to total costs in aggregate across all sampled units, such as the use of existing office supplies by health facility staff.
The sampling strategy employed should aim for internal and external validity of the data Footnote 3 . Sampling strategy should be stated, described, and justified, depending on the workstream and costing objectives. Sampling of different service delivery units is desirable as it provides a more representative picture of costs and highlights cost variation and cost drivers for a strategy or vaccine.
Variation in the cost of the intervention by site/organization, sub-population, or by other drivers of heterogeneity should be explored and reported for retrospective analyses when possible.
The uncertainty around the cost estimates should be appropriately characterized when feasible, (e.g., sensitivity analyses; ranges of results for different input parameter scenarios for cost projections; mean and standard deviation for non-representative samples with multiple units; and confidence intervals or credible intervals for retrospective analyses).
Inclusion and exclusion criteria: “stopping rules” Footnote 4 should be defined, explaining which costs are included and the respective rationale.
Cost estimates should be communicated clearly and transparently to enable decision-makers to interpret and use the results relevant to the original policy and/or programmatic question.
The lack of standardization in terminology, implementation, and principles for vaccine delivery costing has resulted in difficulties in making comparisons among studies, reducing the potential for synthesis of economic evidence across studies for immunization program policy, planning, budgeting, and implementation. As noted earlier, governments need to know the cost of vaccine delivery in order to make decisions on introducing new infant and life-course vaccines, budgeting, and for making improvements in service delivery. The review indicates that existing guidance documents differ somewhat in the inclusion and definitions, of costing terms and costing principles that are recommended, reflecting in part differences in the aims and scope of the costing study.
The review of guidance documents and tools on vaccine delivery costing and iterative discussions among the Working Group members revealed considerable agreement among the different groups working in vaccine delivery costing. Most of the documents made the distinction between economic and financial costs as well as recurrent and capital costs. However, fewer went into detail about the perspective to choose, definition of some costing terms such as start-up costs, micro-costing, and bottom-up/top-down costing, and in some cases, recommended approaches for data collection and analyses. The review also identified gaps in guidance for some analyses, e.g., such as how perspective affects financial costs calculation.
The review revealed that different workstreams focus on distinct aspects of immunization costing with different purposes. These require different types of data collection and analyses. For example, retrospective costing of vaccination focuses on estimating actual resource use, benchmarking of costs, and investigation of variation at the facility and other levels. Cost projections, on the other hand, focus on estimation of (typically incremental) costs to assist in decision-making, preparation of budgets, and evaluating different approaches to a new technology, vaccine, or service delivery strategy.
The process to achieve a consensus statement of vaccine delivery costing methods was facilitated by having extensive consultations with different organizations conducting this work. It also was facilitated by conducting reviews of the guidance documents and costing tools so that similarities, differences, and gaps could be identified. Other strengths of the process include broad and ongoing engagement of experts across various workstreams, including members of the Immunization Economics Community of Practice [ 18 ], as well as dedicated support for facilitation, review, and write-up.
The process to develop a consensus statement provides lessons for developing agreement among other organizations and researchers on types of research methods and tools in other study areas. It requires the potential to bring together organizations working on similar research and then having the time and resources to develop consensus. In addition, it is useful to have some teleconferences and in-person (or virtual meetings with break-out sessions) meetings to have sufficient time to come to consensus.
One limitation of the exercise was that a systematic review was not conducted and some guidelines and costing tools may have been missed. More engagement of country-level practitioners and data and analysis experts outside of those directly involved in the workstreams, as well as a systematic literature search for any methodology documents beyond those known to the workstream participants, would have strengthened the process.
The work on immunization costing is extensive but some gaps were identified. The guidance documents, mostly user manuals for costing tools and the 2002 WHO guidance on introducing new vaccines for cost projections of new vaccines, are not sufficiently detailed regarding data collection and analyses. That is, these do not include instructions on methods of data collection and sampling and analysis methods, when required. Researchers that have piloted the costing tools have also noted that the manuals need to provide more instructions on perspective (see [ 19 ], for example). For example, there is a need for more guidance on how to treat perspective when there is more than one source of financing of vaccines, how to handle slack, etc. As a result, it would be useful to add to current user manuals or develop a new guidance document for cost projections for both single vaccines, multiple vaccines, and immunization programs.
This review and Consensus Statement development process revealed the limitations of our ability to harmonize given that study designs will vary depending upon the policy question that is being addressed and the country context. The Working Group hopes that the consensus statement will contribute to the development of costing guidelines and tools for new vaccines (single or multiple) and immunization programs that are better aligned in terms of definitions, methods, and reporting.
Availability of data and materials
All data generated or analyzed during this study are included in this published article and its additional file.
The experts in the Working Group were from the World Health Organization, UNICEF, US Centers for Disease Control and Prevention, the Bill & Melinda Gates Foundation, Harvard T.H. Chan School of Public Health, International Vaccine Institute, ThinkWell, and the London School of Hygiene and Tropical Medicine.
Two presentations were made: (1) the WHO team’s Ann Levin presented on WHO/IVI/PATH’s work conducting vaccine delivery cost projections with costing tools and the lack of standardization with other workstreams; and (2) ThinkWell’s Annette Ozaltin, representing the BMGF portfolio, presented their work on vaccine costing and the repository of vaccine delivery costs known as the Vaccine Cost Catalogue.
Internal validity refers to the extent of systematic bias in an estimate while external validity is the extent to which the cost estimate can be directly applied to other programmatic setting. (GHCC, pg. A15–A16).
A “stopping rule” defines and explains which costs are included, and how the line is drawn between inclusions and exclusions. (GHCC reference case, pg. B-2)
Abbreviations
2nd Year of Life
Bill & Melinda Gates Foundation
Cervical Cancer Prevention and Control Costing
United States Centers for Disease Control and Prevention
Oral Cholera Vaccine Costing Tool
Comprehensive multi-year plan
- Consensus statement
Expanded Programme on Immunization Costing
Fully immunized person
Global Health Cost Consortium
Immunization Costing Action Network
Information, education, and communication
International Health Economics Association
International Vaccine Institute
Immunization and Vaccines-related Implementation Research Advisory Committee
Low-income country
Middle-income country
Malaria Vaccine Immunization Costing Tool
Seasonal Influenza Immunization Costing Tool
Typhoid Conjugate Vaccine Costing Tool
Vaccine Technology Costs and Health Impact Assessment Tool
World Health Organization
Johns Hopkins University, International Vaccine Access Center. Methodology report: decade of vaccines economics (DOVE). Return on investment analysis. Medford: Immunization Economics; 2019. https://static1.squarespace.com/static/556deb8ee4b08a534b8360e7/t/5d56d54c6dae8d00014ef72d/1565971791774/DOVE-ROI+Methodology+Report+16AUG19.pdf . Accessed March 2020.
Google Scholar
WHO. Immunization agenda 2030: a global strategy to leave no one behind; 2019.
Vaughan K, Ozaltin A, Moi F, Griffiths UK, Mallow M, Brenzel L. 2020 reporting gaps in immunization costing studies: recommendations for improving the practice. Vaccine X. 2020;5:100069.
Article Google Scholar
WHO 2018. Immunization and vaccine-related implementation research advisory committee (IVIR-AC): executive summary, 6-8 march 2018, vol. 93. Chamonix: WHO Weekly Epidemiological Record, No 24; 2018. p. 345–56.
WHO 2019. Immunization and Vaccine-related Implementation Research Advisory Committee (IVIR-AC) recommendations. WHO Wkly Epidemiol Record No 24. 2019;94:225–32.
Immunization Costing Action Network (ICAN). Immunization delivery costs in low- and middle-income countries: a methodology note for the systematic review, cost catalogue, and analytics. Washington, DC: ThinkWell; 2018.
WHO, 2020. Immunization and Vaccine-related Implementation Research Advisory Committee (IVIR-AC): summary and recommendations, September 2019. WHO Wkly Epidemiol Record No 49. 2020;95:609–28.
Vassall A, Sweeney S, Kahn J, Gomez G, Bollinger L, Marseille E, et al. Reference case for estimating the costs of Global Health services and interventions; 2017.
Husereau D, Drummond M, Petrou S, Carswell C, Moher D, Greenberg D, et al. Consolidated health economic evaluation reporting standards (CHEERS) statement. BMJ. 2013;346:f1049. https://doi.org/10.1136/bmj.f1049 .
Article PubMed Google Scholar
ImmunizationEconomics.org. 2021. EPIC projects. The Bill & Melinda Gates Foundation. https://immunizationeconomics.org/epic . Accessed 24 Jan 2022.
Brenzel L. 2013. Common approach for the costing and financing analyses of routine immunization and new vaccine introduction costs. Working Paper. Bill & Melinda Gates Foundation.
Harvard T. H. Chan School of Public Health. 2019. How to cost immunization programs: a practical guide on primary data collection and analysis.
International Vaccine Institute and WHO, Choltool: Planning and Costing User’s Guide. Seoul, South Korea.
WHO. Guidelines for estimating costs of introducing new vaccines into the national immunization system. Geneva: Switzerland; 2002.
WHO, WHO Cervical Cancer Prevention and Control Costing Tool (C4P) User’s Guide. Geneva, Switzerland. https://cdn.who.int/media/docs/default-source/immunization/hpv/who-cervical-cancer-prevention-and-control-costing.pdf?sfvrsn=b56c33cb_7 . Accessed 1 Feb 2022.
World Health Organization. Immunization Costing & Financing: a tool and user guide for comprehensive multi-year planning (cMYP). Geneva: WHO; 2006.
WHO, WHO Seasonal Influenza Immunization Costing Tool: Planning and Costing User’s Guide. Geneva, Switzerland.
ImmunizationEconomics.org. 2021. Community of practice. The Bill & Melinda Gates Foundation. https://immunizationeconomics.org/community . Accessed 24 Jan 2022.
Pallas S, Ahmeti A, Morgan W, Preza I, Nelaj E, Ebama M, et al. Program cost analysis of influenza vaccination of health care workers in Albania. Vaccine. 2020;38(2):220–7. https://doi.org/10.1016/j.vaccine.2019.10.027.Epub 2019 Oct 25.
Download references
Acknowledgements
We thank Xiao Xian Huang and Stéphane Verguet, two Working Group members, for their contributions to and review of the consensus statement. We also thank Shuoning Huang for her coordination and contribution to the first draft of the consensus statement. We acknowledge comments received on earlier versions of the consensus statement from Taiwo Abimbola, Anna Hidle, Timothy Brennan, Nelly Mejia, and Carlo Davila Payan of the CDC.
The views in this manuscript are those of the authors in their individual capacities and do not represent the official positions of the authors’ organizations.
The WHO provided funding for the consultant that led the working group.
Author information
Laura Boonstoppel, Logan Brenzel, Ulla Griffiths, Raymond Hutubessy, Mark Jit, Vittal Mogasale, Sarah Pallas, Stephen Resch, Christian Suharlim, and Karene Hoi Ting Yeung contributed equally and are listed in alphabetical order.
Authors and Affiliations
Levin & Morgan LLC, Bethesda, USA
ThinkWell, Geneva, Switzerland
Laura Boonstoppel
Bill & Melinda Gates Foundation, Seattle, USA
Logan Brenzel
UNICEF, New York, USA
Ulla Griffiths
Department of Immunization, Vaccines and Biologicals, World Health Organization, Geneva, Switzerland
Raymond Hutubessy & Karene Hoi Ting Yeung
London School of Hygiene & Tropical Medicine, London, UK
International Vaccine Institute, Seoul, South Korea
Vittal Mogasale
Centers for Disease Control and Prevention, Atlanta, USA
Sarah Pallas
Harvard T.H. Chan School of Public Health, Boston, USA
Stephen Resch & Christian Suharlim
You can also search for this author in PubMed Google Scholar
Contributions
AL reviewed the guidance documents and costing tools and was the lead author. All authors contributed to the data interpretation and critical revision of the manuscript. All authors read and approved the final manuscript.
Corresponding author
Correspondence to Raymond Hutubessy .
Ethics declarations
Ethics approval and consent to participate.
Not applicable.
Consent for publication
Competing interests.
The authors declare that they have no competing interests.
Additional information
Publisher’s note.
Springer Nature remains neutral with regard to jurisdictional claims in published maps and institutional affiliations.
Supplementary Information
Additional file 1. .
Consensus Statement on Vaccine Delivery Costs which includes a review of vaccine delivery cost guidance documents and costing tools as well as a consensus statement on the terminology and methodological principles to be used for vaccine delivery costing. It includes two figures and 6 tables. Figure S1. Major current workstreams in vaccine delivery costing identified by working group. Figure A1 – Timeline for developing a Consensus Statement on Vaccine Delivery Costs. Table A2a. List of guidelines by publication year, target interventions, and purposes. Table A2b. List of costing tools for vaccine delivery or immunization program. Table A3. Definitions of costing terms in guidance documents. Table A4. Comparison of costing principles among guidance. Table A5. Characteristics of costing workstreams. Table 6A. Data sources, sampling and characterization of uncertainty, and terminology by workstreams.
Rights and permissions
Open Access This article is licensed under a Creative Commons Attribution 4.0 International License, which permits use, sharing, adaptation, distribution and reproduction in any medium or format, as long as you give appropriate credit to the original author(s) and the source, provide a link to the Creative Commons licence, and indicate if changes were made. The images or other third party material in this article are included in the article's Creative Commons licence, unless indicated otherwise in a credit line to the material. If material is not included in the article's Creative Commons licence and your intended use is not permitted by statutory regulation or exceeds the permitted use, you will need to obtain permission directly from the copyright holder. To view a copy of this licence, visit http://creativecommons.org/licenses/by/4.0/ . The Creative Commons Public Domain Dedication waiver ( http://creativecommons.org/publicdomain/zero/1.0/ ) applies to the data made available in this article, unless otherwise stated in a credit line to the data.
Reprints and permissions
About this article
Cite this article.
Levin, A., Boonstoppel, L., Brenzel, L. et al. WHO-led consensus statement on vaccine delivery costing: process, methods, and findings. BMC Med 20 , 88 (2022). https://doi.org/10.1186/s12916-022-02278-4
Download citation
Received : 18 October 2021
Accepted : 27 January 2022
Published : 08 March 2022
DOI : https://doi.org/10.1186/s12916-022-02278-4
Share this article
Anyone you share the following link with will be able to read this content:
Sorry, a shareable link is not currently available for this article.
Provided by the Springer Nature SharedIt content-sharing initiative
- Delivery cost
- Immunization
BMC Medicine
ISSN: 1741-7015
- Submission enquiries: [email protected]
- General enquiries: [email protected]
Thank you for visiting nature.com. You are using a browser version with limited support for CSS. To obtain the best experience, we recommend you use a more up to date browser (or turn off compatibility mode in Internet Explorer). In the meantime, to ensure continued support, we are displaying the site without styles and JavaScript.
- View all journals
- My Account Login
- Explore content
- About the journal
- Publish with us
- Sign up for alerts
- Review Article
- Open access
- Published: 10 April 2024
Insights into vaccines for elderly individuals: from the impacts of immunosenescence to delivery strategies
- Yingying Hou ORCID: orcid.org/0009-0001-0561-5835 1 , 2 na1 ,
- Min Chen 1 , 2 na1 ,
- Yuan Bian 1 , 2 na1 ,
- Yuan Hu 1 , 2 ,
- Junlan Chuan 1 , 2 ,
- Lei Zhong ORCID: orcid.org/0000-0002-1901-9698 1 , 2 ,
- Yuxuan Zhu ORCID: orcid.org/0000-0002-5234-8506 1 , 2 &
- Rongsheng Tong ORCID: orcid.org/0000-0002-9525-9159 1 , 2
npj Vaccines volume 9 , Article number: 77 ( 2024 ) Cite this article
250 Accesses
7 Altmetric
Metrics details
- Pharmaceutics
Immunosenescence increases the risk and severity of diseases in elderly individuals and leads to impaired vaccine-induced immunity. With aging of the global population and the emerging risk of epidemics, developing adjuvants and vaccines for elderly individuals to improve their immune protection is pivotal for healthy aging worldwide. Deepening our understanding of the role of immunosenescence in vaccine efficacy could accelerate research focused on optimizing vaccine delivery for elderly individuals. In this review, we analyzed the characteristics of immunosenescence at the cellular and molecular levels. Strategies to improve vaccination potency in elderly individuals are summarized, including increasing the antigen dose, preparing multivalent antigen vaccines, adding appropriate adjuvants, inhibiting chronic inflammation, and inhibiting immunosenescence. We hope that this review can provide a review of new findings with regards to the impacts of immunosenescence on vaccine-mediated protection and inspire the development of individualized vaccines for elderly individuals.
Similar content being viewed by others
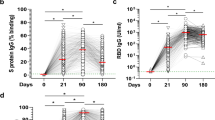
Lower vaccine-acquired immunity in the elderly population following two-dose BNT162b2 vaccination is alleviated by a third vaccine dose
Laurent Renia, Yun Shan Goh, … Lisa F. P. Ng
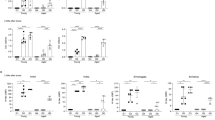
Effective inactivated influenza vaccine for the elderly using a single-stranded RNA-based adjuvant
Yoo-Jin Bang, So-Hee Hong, … Jae-Hwan Nam
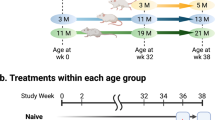
mRNA booster vaccination protects aged mice against the SARS-CoV-2 Omicron variant
Etsuro Nanishi, Marisa E. McGrath, … David J. Dowling
Introduction
Aging is a common, inevitable, and complex process in nature 1 , and the aging population is a worldwide concern. Currently, the proportion of elderly individuals is rapidly increasing worldwide. By 2050, the global population over 60 years old will increase to 2.1 billion 2 . Aging is accompanied by a decline in physiological function and progressive immune system degradation, which increases the risk and severity of infectious diseases, malignant tumors, and various chronic diseases. In particular, patients who died from the novel coronavirus disease 2019 (COVID-19) infection were mainly older individuals suffering from underlying conditions 3 , such as obesity, heart disease, cancer, hypertension or high blood pressure, lung disease, and diabetes. Therefore, the public health service and healthcare systems are facing severe challenges caused by aging.
Vaccination is one of the most important successes of modern medicine and is a powerful weapon for preventing and treating fatal infectious diseases 4 . Over the years, vaccines targeting multiple pathogens have saved hundreds of millions of lives 5 , 6 . People are increasingly aware of the great potential of vaccines in controlling disease outbreaks and protecting older people. Individuals over 65 years are recommended to receive vaccines to prevent severe diseases, such as influenza, herpes zoster, pneumococcal disease, respiratory syncytial virus infection, tetanus, and diphtheria 7 . Nevertheless, the vaccination efficiency in elderly individuals is often reduced due to immunosenescence 8 . For example, the capacity of influenza vaccines to induce immune protection is age-related, with efficacy ranging from 70% to 90% in young people but decreasing to 30–50% in people over 65 years 9 .
The concept of immunosenescence was first proposed by ref. 10 . Subsequently, scientists began to deeply study the mechanism and role of immunosenescence to find key biomarkers that could guide the prevention and treatment of aging-related diseases. Immunosenescence is a complex process that involves organ reorganization and numerous regulatory processes at the cellular level. The decline in the amount and function of immune cells results in impaired immune responses. Therefore, elderly individuals are at the greatest risk of infection and the most challenging to protect by vaccination 11 . With the aging of the global population and the increased risk of epidemics, the research and development of adjuvants and vaccine systems that offer high-efficacy immune protection for elderly individuals is key for healthy global aging and severe disease prevention. Extensive knowledge of immunosenescence, its impact on vaccination, and its immunological mechanisms is needed to provide scientific insights to optimize vaccines for elderly individuals.
In this review, we analyzed alterations mediated by immunosenescence at the cellular and molecular levels and reviewed emerging strategies to boost the potency of vaccines in elderly individuals, including increasing the antigen dose, preparing multivalent antigen vaccines, adding appropriate adjuvants, inhibiting chronic inflammation, and inhibiting immunosenescence. The prospects and challenges of the various design strategies are also discussed. We hope to incite deep interest in individualized vaccines and provide innovative thoughts to improve vaccines for elderly individuals.
Impact of immunosenescence on vaccine efficacy
Vaccination capacity is often evaluated based on the level of antigen-specific antibodies in sera, especially antibodies with neutralizing ability, which reflects the immediate responses to pathogens. The memory B cells that are formed through vaccination are also crucial and are quickly activated, expanded, and secrete effector antibodies when confronted with a pathogen reinfection. Meanwhile, in chronic viral infections and tumors, there is an increasing amount of focus on the role of CD4 + and CD8 + T cell responses 6 , 12 ; these T cells secrete effector molecules that can inhibit pathogens directly or indirectly.
The immunological and molecular signatures of elderly individuals are different from those of young individuals. A study on the comprehensive characteristics of immune responses in the elderly (≥65 years old) showed that those who did not respond to the influenza vaccine presented with multiple states of immune inhibition 13 . In general, the number and function of naïve B and T cells in older individuals are reduced, resulting in weakened immunity to neo-antigens. Studies have shown that aging immune cells can generate a sufficient primary antibody response, although at a slower rate and with a lower ability to neutralize pathogens 14 . Meanwhile, the CD4 + and CD8 + T cell responses are also diminished 15 .
Our group has previously proposed that the spatial delivery of subunit vaccines should undergo an in vivo cascade that involves the following six steps: lymph node targeted delivery, dendritic cell (DC) subset targeting, B cell modulation, antigen uptake, antigen cross-presentation, and antigen-presenting cell (APC) regulation to stimulate T cells 6 . All of these immune steps are impacted during the aging process, thereby altering immunity. The age-associated alterations in lymph nodes, DCs, B cells, and T cells are summarized in Fig. 1 .
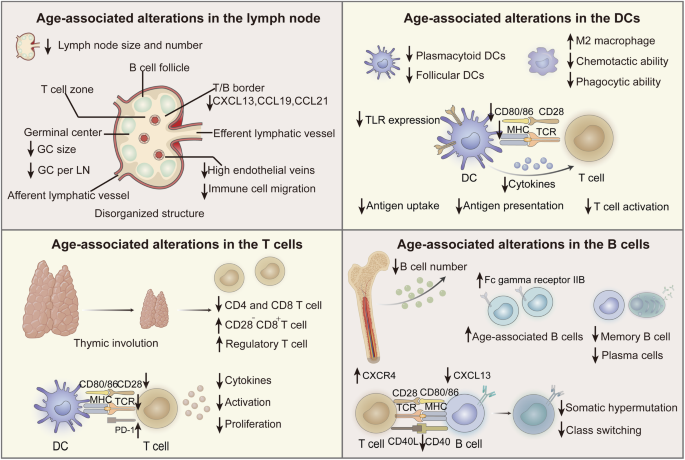
GC germinal center, LN lymph node, DC dendritic cell, MHC major histocompatibility complex, TCR T cell receptor, TLR toll-like receptor, PD-1 programmed death receptor-1. (Created with Adobe illustrator and BioRender.com.).
The lymph node contains plentiful immune cells and is the main location where APCs present antigens to activate T cells, leading to the development of adaptive immune responses 16 . Targeting lymph nodes will induce faster and more direct interactions between vaccines and immune cells, which can lead to effective immune activation. Both the size and number of lymph nodes are reduced with aging. The crucial site of the humoral immune response in lymph nodes is the germinal center (GC). However, the GC area and the intensity of the GC response decrease with age 17 , 18 . At the same time, the decrease in endothelial veins in lymph nodes and the reduced phosphoinositide 3-kinase and CC-chemokine receptor 7 signals on immune cells limit the migration of immune cells to lymph nodes 19 , 20 . This change may ultimately lead to weakened lymph node targeting ability.
DCs are critical APCs that initiate naïve T cells, which play a crucial role in bridging innate and adaptive immunity 21 . DCs are divided into several important subgroups: conventional DCs mainly serve to prime naïve T cells; plasmacytoid DCs (pDCs) can produce large amounts of type I interferon (IFN) to participate in antiviral immunity; monocyte-derived dendritic cells (moDCs) can present antigens to effector T cells and secrete cytokines; Langerhans cells (LCs) are epidermal DCs that can present antigens to induce T cell immunity 22 . In addition, follicular DCs are specialized subsets in the lymphoid follicles that promote B cell antigen presentation 23 . The frequencies of circulating pDCs and follicular DCs decrease, while the number of circulating moDCs remains unchanged with age 8 . After being internalized by APCs, the antigen is processed into a peptide. Then, the antigen peptide and major histocompatibility complex (MHC) molecules generate the first signal presented to T cells. During this process, APCs gradually mature to express costimulatory molecules (the second signal) and secrete cytokines (the third signal). These cytokines, such as interleukin (IL)-12, IL-4, and IL-6, assist in the polarization of T cells into Th1, Th2, or Th17 cells. As age increases, the overall number of lymphocytes decreases and transfers toward myeloid cells. As such, there is a decrease in the number of DCs with increasing age 24 . The expression of toll-like receptor (TLR) 3, 7/8, costimulatory molecules, and MHC molecules on DCs decreases with age 8 . After stimulation with TLR7 or TLR9, the pDCs of elderly individuals also show reduced secretion of tumor necrosis factor (TNF) -α and IFN-α 25 . Therefore, the phagocytosis, antigen presentation, and cytokine secretion abilities of DCs decrease, which further hinders subsequent T cell activation and immune responses. Furthermore, distinct vaccines have been shown to stimulate different subsets of DCs 26 . Identifying the diverse impacts of immunosenescence on DC subsets is pivotal for developing vaccines for older individuals. Macrophages serve as key APCs; with age, macrophages have impaired chemotactic and phagocytic abilities. In older mice, a high frequency of M2 macrophages was observed in the spleen and lymph nodes, which have abilities of angiogenesis and tumor growth 27 . The researchers proposed that macrophages from older mice are particularly sensitive to signals that promote their development into highly immunosuppressive M2 macrophages, which promote tumor development. IL-2/anti-CD40 antibody immunotherapy to activate macrophages can rescue age-related T cell dysfunction. Similarly, Prieto et al. found that senescent alveolar macrophages counteract cytotoxic T-cell accumulation to promote lung tumorigenesis 28 . Surprisingly, therapeutic interventions targeting senescent macrophages by knocking out their specific expression molecules can delay tumor progression. These observations may inspire the design of cancer vaccines for elderly individuals. M2 macrophages can be targeted for removal by specific expression molecules, or their polarization and immunosuppression can be corrected to improve the efficacy of vaccines in the elderly.
B cells are the center of humoral immunity and can be activated, amplified, and secrete protective antibodies that respond to immunization. At the same time, B cells are also APCs that can present antigens following their recognition via surface of B cell receptors 29 . The bone marrow is the main source of B cells. With increasing age, the hematopoietic function of the bone marrow decreases, which leads to a decline in the number of B cells. Studies have shown that the number of naïve B cells decreases with age, while memory B cells generally remain stable 30 . However, Pritz et al. found that the frequency of naïve B cells remains unchanged or increases with age, and the number of plasma cells and memory B cells decreases 8 , 31 . This discrepancy could be due to the use of different markers to define B cell subgroups. Additionally, differences in the age groups and cohort sizes of the analyzed samples may also affect the study results. At the same time, the age-associated B cells (ABCs) in both mice and humans are antigen-experienced memory B cells generated during microbial infections and vaccinations 32 . Aging mice showed an increased number of ABCs, which secrete cytokines to impair the generation of young pro-B cells 18 . Yam-Puc et al. reported that increased ABC frequency in humans is associated with reduced antigen-specific memory B cells and decreased neutralizing capacity against SARS-CoV-2 33 . They also identified that increased expression of the inhibitory receptor Fc gamma receptor IIB can bind immune complexes to enhance their clearance, contributing to reduced vaccine response.
The GC is polarized into two functionally distinct regions: the light and dark zones. In the light zone, the B cells capture antigens and present them to the T follicular helper (Tfh) cells. In the dark region, the B cells proliferate and mutate, improving the specificity and affinity of the antibodies. Tfh cells through the CXCR5/CXCL13 recognition position to the light zone of the GC and through the CXCR4/CXCL12 recognition position to the dark zone of the GC 34 . CXCL13 is a chemokine in B cell follicles that is pivotal for transporting Tfh CD4 cells to B cell follicles during the humoral immune response 35 . The level of CXCL13 also decreases with age 36 . Immunosenescence reduces naïve CD4 T cell differentiation into Tfh cells, so there is an insufficient amount of T cells to assist the B cell response 37 . Silva-Cayetano et al. found that the expression of CXCR4 increased in Tfh cells from aged mice, while the expression of CXCR5 did not change 38 . The increased expression of CXCR4 led to enhanced chemotaxis of CXCL12 in the dark zone of GC, directly decreased the amounts of antigens presented to B cells, and decreased specific antibody responses. Moreover, research has shown that the expression of activation-induced cytidine deaminase necessary for affinity maturation and class switching in B cells is significantly reduced in elderly individuals 39 , which subsequently leads to decreased affinity and antibody responses. However, Stiasny et al. found that vaccination against tick-borne encephalitis can induce high avidity of antibodies and functional activity even in the elderly 40 . These alterations in B cells may explain the impairment in protective humoral immunity in older individuals after vaccination.
As the key effector cells of immune responses, T cells are also impacted by aging more than other cells. The replenishment of T cell frequency is achieved through export by the thymus and the self-renewal of peripheral naïve T cells 41 . There is a loss of naïve T cells in elderly individuals caused by thymic involution. Moreover, the expression of the T cell receptor (TCR) and costimulatory molecules CD27 and CD28 decreases, which inhibits T cell stimulation and proliferation in elderly individuals following vaccination 8 . The lack of CD27 and CD28 expression in older individuals is a feature of T cell senescence, presumably caused by repeated T cell activation. The mechanism underlying the reduced expression of CD27 and CD28 deserves further research; this information could be used to design methods to restore T cell function and improve immunity in elderly individuals. Meanwhile, the expression of programmed death receptor-1 (PD-1) on T cells increases with age, while the amount of CD28 - CD8 + T cells and regulatory T (Treg) cells that inhibit T cell activation and proliferation increases 7 , 8 . In particular, CD28 - CD8 + T cells inhibit T cell activation and expansion by producing TGF-β, IL-7, and IL-10 42 . T cells with high expression of PD-1 inhibit T cell activity through the PD-1/programmed death ligand 1 (PD-L1) pathway, thereby inhibiting the ability of T cells to kill tumor cells in the tumor microenvironment and allowing the immune escape of tumors 43 . Thus, these changes in senescent T cells play an essential role in the reduced immune response following vaccination. The deficiency of CD4 + T cells in older individuals also markedly affects immunity. Tfh cells are a subgroup of CD4 + T cells, which helps antigen-specific B cell proliferation, class switching, and somatic hypermutation 44 . Compared with young individuals, the number of circulating Tfh (cTfh) cells is markedly reduced in elderly individuals. In addition, coculture of cTfh cells from older individuals with allogeneic naïve B cells from a young subject led to impaired expansion and reduced antibody production 45 . Therefore, strategies to enhance specific Tfh cell stimulation could improve vaccine-induced immunity.
Inflammation and immunity
Immunosenescence is accompanied by the development of a chronic and systemic sterile inflammatory environment called inflammaging 46 . The characteristic factors in this environment include C-reactive protein, TNF-α, IL-6, and other proinflammatory cytokines; the levels of these factors are higher in older adults than in young people 47 . The acute inflammatory reaction is essential in triggering immunity against invasive pathogens. After initial inflammation, the amount of inflammation is reduced for a period to avoid continuous tissue damage and restore tissue homeostasis 48 . Nevertheless, research has shown that chronic inflammation negatively impacts immunity because increases in the inflammatory response reduce the effectiveness of vaccines 49 .
Chronic inflammation during aging is caused by multiple mechanisms. Chronic viral infections, such as cytomegalovirus, can induce lifelong latent infections after the initial infection, periodic reactivation of the virus, and the initiation of subclinical immunity 50 . During aging, the proportion of muscle decreases and visceral fat increases. Obesity, especially visceral fat accumulation, can induce inflammatory sites that infiltrate monocytes, phagocytes, B cells, and T cells, which produce inflammatory cytokines 51 . Gut permeability increases with age, which causes the leakage of intestinal contents into the bloodstream. For example, lipopolysaccharides in circulation can trigger inflammatory cytokine secretion by monocytes via pattern recognition receptor (PRR) activation 52 . Moreover, changes in the microbiome in elderly individuals may also elevate the level of circulating inflammatory cytokines 53 .
Damage-associated molecular patterns are cellular products that are released during stress, injury, or death and include calcium-binding proteins, histones, ATP, uric acid, and heparin sulfate 49 . When faced with acute inflammation, there is a decrease in efferocytosis and the clearance of apoptotic neutrophils during the regression phase of inflammation. Therefore, the ability to resolve inflammation in elderly individuals is weakened compared to that of young individuals 54 . Meanwhile, senescent cells secrete multiple inflammatory cytokines (such as IL-1β, IL-6, and TNF-α), chemokines (such as CCL2 and IL-8), and growth factors. This secretion of proinflammatory mediators is called the senescence-associated secretory phenotype (SASP), which contributes to the inflammatory microenvironment 55 . In addition, the signaling pathway of cyclic GMP-AMP synthase and stimulator of interferon genes (STING) plays a key role in the development of chronic inflammation and functional decline during aging 56 . The NOD-, LRR- and pyrin domain-containing protein 3 (NLRP3) inflammasomes, which promote the maturation and release of IL⁃1β and IL⁃18, are involved in a variety of host immune and inflammatory responses. Overactivation of the NLRP3 inflammasome can lead to pathological inflammation and is associated with age-related diseases as it is involved in the inflammatory aging process 57 . The nuclear factor kappa-light-chain-enhancer of activated B cells (NF-κB) is a family of transcription factors that also play an essential role in mediating immune and inflammatory responses 8 .
Inflammatory cytokines can increase the expression of inhibitory ligands on immune cells to alter immunity. For example, TNF-α facilitates the expression of PD-L1 on APCs. PD-L1 binds to PD-1 expressed in T cells 58 ; and the significantly increased level of PD-1 on senescent T cells inhibits the immune response 59 . Inflammation-related cytokines may also increase the frequency and promote the function of Foxp3 + Treg cells in elderly individuals; these cells are recruited to inflammatory sites and inhibit antigen-specific immune responses 60 . In addition, SASP components impact the immune response in various ways. Monocyte-derived and plaque-infiltrating macrophages in elderly patients with coronary artery disease express PD-L1 with high surface density, resulting in suppressed T cell immunity 61 . Prostaglandin E2 can impair the cytotoxic T lymphocyte (CTL) response and suppress CTL survival 62 .
Molecular hallmarks of aging and immunity
Recently, important advances have been made in research on signaling pathways and molecular mechanisms that influence senescence. Researchers have proposed certain molecular hallmarks of aging (Fig. 2 ), such as genomic instability, telomere attrition, epigenetic alterations, loss of proteostasis, compromised autophagy, and mitochondrial dysfunction 63 , 64 . Considering our background knowledge of vaccine-induced immune responses, we speculate that compromised autophagy, the loss of proteostasis, and telomere attrition may affect the immune responses induced by vaccines.
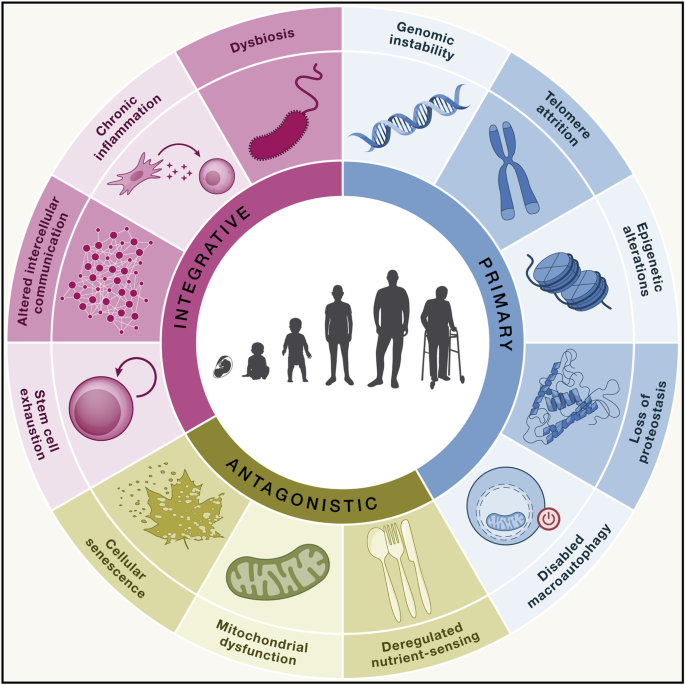
Copyright 2023, Cell Press.
Autophagy activity decreased in different tissues of different species with age. Autophagy gene transcripts, such as ATG5, ATG-7, and BECN1, decreased with age 65 . Recent findings support the idea that the reduction in autophagy is the core molecular mechanism underlying immunosenescence 66 . Autophagy is a highly conserved process that can degrade defective intracellular organelles and misfolded protein aggregates 67 . Autophagy can prolong life by inhibiting the mammalian target of rapamycin (mTOR) signaling pathway or activating the adenosine 5′-monophosphate-activated protein kinase (AMPK) pathway 68 . In aging, damaged proteins and organelles gradually accumulate. Cell quality control systems are dysfunctional, such as the unfolded protein response and autophagy, resulting in the reduced antigen presentation by APCs 69 . Wang et al. conjugated poly (β-amino ester) with an autophagy-inducing peptide to self-assemble a nanovaccine 70 . The results showed that improving antigen cross-presentation and the vaccine-induced immune response are promoted by autophagy in DCs. Autophagy can lead to the formation of antigen storage compartments in APCs, prolong the allowed storage time of antigens, and facilitate antigen presentation and subsequent initiation of CD8 + T cell reponses 71 . In addition, autophagy is pivotal in regulating T cell responses. The loss of autophagy results in reduced T cell survival, proliferation, and cytokine secretion following TCR activation 72 .
Spermidine is a natural polyamine that can improve the health and extend the lifespan of entire species 73 . Spermidine, as an autophagy agonist 74 , is undergoing clinical trials to investigate its ability to restore autophagy in T and B cells to improve vaccine potency in elderly individuals (NCT05421546). Metformin is a drug used to treat type 2 diabetes safely and can be used for children and even pregnant women. Metformin can induce cell autophagy by activating the AMPK signaling pathway 75 , inhibiting the stimulation of the NLRP3 inflammasomes, and improving aging-related inflammatory rresponses 76 . A completed clinical trial verified that metformin can elicit more effective immune responses in elderly individuals given the influenza vaccine (NCT03996538). Another study also investigated whether metformin can improve immune responses to the pneumococcal conjugate vaccine in older individuals (NCT03713801). Future research may focus on utilizing spermidine or metformin in vaccine design to elevate immunity in elderly individuals.
With extensive research on the molecular mechanism of aging, it has been found that telomeres stimulate molecular pathways that drive the aging process and related diseases 77 . Telomeres are a group of repetitive sequences that protect the ends of chromosomes and prolong the cellular lifespan. As the clock of life, telomeres gradually shorten with cell division and aging. Telomerase can prolong telomere repair to alleviate telomere loss caused by T cell proliferation, but telomerase activation is insufficient in protecting T cells from immunosenescence. Recent studies have shown that APCs form immunological synapses with TCRs through MHC and present antigens to T cells. T cells elongate their telomeres by acquiring telomeres in extracellular vesicles (EVs) from APCs 78 . In vivo experiments in mice found that telomere-containing EVs can elicit antigen-specific T cell expansion, stem cell-like memory T cells, and central memory cell proliferation. Additionally, a comparative experiment using the influenza vaccine indicated no significant difference in the short-term protection of the vaccine based on telomere EVs. However, the long-term protection of the telomere EV group was dramatically better than that of the group without telomere EVs. This finding further showed that telomere-containing EVs improved immune memory and long-lasting immunity. The results of this study suggest that vesicles carrying telomeres may be a potential strategy for inhibiting T cell immunosenescence and provide new ideas for the design of vaccines for elderly individuals.
We think that the identification of new markers of immunosenescence will inspire vaccine design and development in the future. In conclusion, we summarized the alterations in immunosenescence at the systematic, cellular, and molecular levels in the elderly (Fig. 3 ).
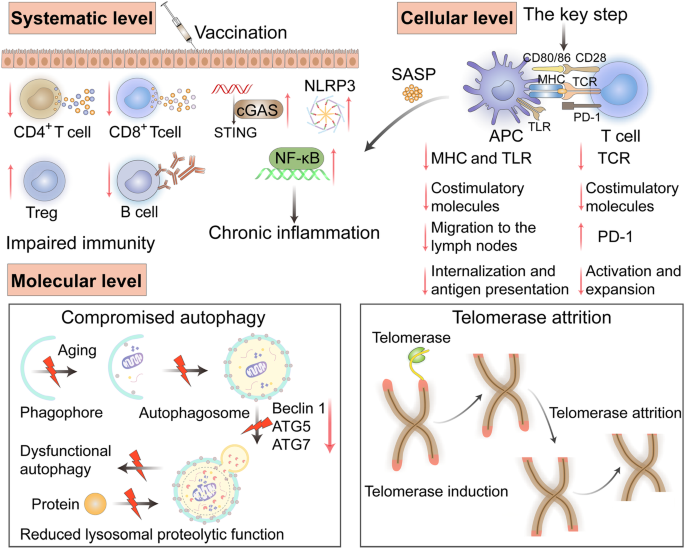
The generation and maintenance of protective immunity induced by vaccination are weakened. The cGAS-STING, NLRP3 and NF-κB signaling pathways were upregulated, leading to chronic inflammation. Meanwhile, the expression of MHC, TLRs, and costimulatory molecules on APCs is reduced, leading to reduced migration to the lymph node, internalization, and antigen presentation of APCs. T cells have low expression of TCRs and costimulatory molecules, but high expression of PD-1, which leads to reduced T cell stimulation. At the molecular level, there are mainly compromised autophagy and telomere attrition. STING stimulator of interferon genes, cGAS cyclic GMP-AMP synthase, NLRP3 NOD-, LRR- and pyrin domain-containing protein 3, NF-κB nuclear factor kappa-light-chain-enhancer of activated B cells, SASP senescence-associated secretory phenotype, APC antigen-presenting cell, MHC major histocompatibility complex, TCR T cell receptor, TLR toll-like receptor, PD-1 programmed death receptor-1, ATG5 autophagy-related 5, ATG7 autophagy-related 7. (Created with Adobe illustrator).
Design strategies for vaccines for elderly individuals
Although the vaccine-induced immunity of elderly individuals is significantly reduced, some strategies are still available to enhance protective immunity. Understanding what strategies already exist in this field is imperative for the development of other high-efficacy vaccines for elderly individuals.
High-dose vaccines
Compared with traditional vaccines, a high-dose influenza vaccine can provide four times the dose of hemagglutinin antigen and improve the immunogenicity and vaccine potency in people ≥65 years old 79 . High-dose influenza vaccine immunization generates a higher number of antibodies and improves protection against influenza virus in elderly individuals 80 . At the same time, the risk of influenza virus infection, hospitalization, and mortality among older people has significantly reduced 81 . We speculate that an increased antigen dose will increase immunogenicity and induce long-lasting T cell and B cell responses. With age, the quantity of naïve T cells and the diversity of TCRs continue to decline 82 , which means that the possibility of naïve T cells meeting the homologous antigen presented by APCs after vaccination is reduced. However, increasing the vaccine dose can help compensate for the decrease in naïve T cells by increasing the density of DCs that present antigens to T cells in secondary lymphoid tissues, which boosts the possibility of productive interactions between T cells and APCs.
From the perspective of delivery carriers, microparticles can encapsulate more antigens than nanoparticles. Studies have reported that particles with large surface areas, such as metal-organic frameworks, mesoporous silica, and Pickering emulsions, have excellent antigen-loading capabilities 83 , 84 , 85 . Moreover, some antigen self-assembled nanoparticles can achieve high-dose antigen delivery without additional carriers 86 , 87 . These delivery carriers may be useful in high-dose vaccines.
Multivalent vaccines
An ongoing challenge in vaccination is developing a vaccine that can boost broad protective immunity 88 . Multivalent vaccines are an excellent choice and have proven effective in practice. Multivalent vaccines contain antigens from a variety of strains/serotypes of pathogens, which can produce cross-protection and provide broader coverage of antigenic variable pathogens 89 . Commercially available pneumococcal 13-valent and 23-valent vaccines contain multiple antigen subtypes that can protect against the common serotypes of Streptococcus pneumoniae in older adults 90 , 91 . A comparison of trivalent and monovalent influenza vaccines showed that polyvalent vaccines can evoke broader coverage of heterotypic and cross-protection 92 .
When developing multivalent vaccines, it is first necessary to select a combination of antigens with diverse valence types to ensure their immunogenicity. Subsequently, studies should focus on evaluating whether multivalent vaccines can induce more neutralizing antibodies and cross-neutralization against other viral variants, thereby verifying whether broader protection is achieved.
Adjuvanted vaccines
Adjuvants are substances that can improve vaccine efficacy through versatile mechanisms, such as promoting proinflammatory cytokine secretion, recruiting and stimulating innate immune cells, activating PRRs expressed on immune cells, and promoting antigen presentation 93 , 94 . Adding appropriate adjuvants can decrease the required dosage of antigens, reduce the number of vaccinations needed, and broaden immune protection 95 ; adjuvant research is an important direction for improving vaccine efficiency. A scoping review showed that several adjuvants such as MF59, AS03, AS01, and CpG oligodeoxynucleotides (CpG-ODN) are effective in older adults 96 . We will present these adjuvants next.
The Fluad® influenza vaccine contains an oil-in-water emulsion MF59 (an oil-in-water emulsion of squalene oil), which was licensed in 1997 in Italy, and is effective in elderly individuals 97 . The addition of MF59 adjuvant reduces the rate of infection and hospitalization of elderly individuals with influenza virus 97 . Studies have shown that the MF59 adjuvant can significantly increase the number of influenza virus antigen-specific antibodies and the serum conversion rate compared to vaccination with no adjuvant 98 , 99 . MF59 can induce the secretion of cytokines and chemokines, recruit immune cells such as monocytes and neutrophils to the injection site, and promote the transport of antigens to lymph nodes 93 . The oil-in-water emulsion AS03, which was used in another influenza vaccine, also showed high immunogenicity in the elderly 97 . AS03 contains α-tocopherol components, which strongly upregulate the expression of inflammatory cytokines and chemokine coding genes in the lymph nodes 100 . AS03 can also activate the CD4 + T cell response, thus leading to the continuous production of neutralizing antibodies and more memory B cells 101 . Additionally, the safety and immunogenicity of recombinant influenza vaccines using matrix M-1 saponin complex adjuvants have been studied in older people (NCT03293498). Another adjuvant, AS01, is a liposome-based vaccine adjuvant system that uses saponin QS21 and the TLR4 agonist monophosphoryl lipid A (MPLA) 102 ; vaccines using this system showed improved humoral and cellular immunity. MPLA can directly activate APCs through TLR4 activation, thereby stimulating costimulatory molecule expression and cytokine secretion. QS-21 activates caspase-1 in subcapsular sinus macrophages and activates cytotoxic CD8 + T cells 103 . These two components simultaneously act on the innate immune system to synergistically amplify the immune response. An adjuvanted recombinant herpes zoster vaccine also includes AS01 and glycoprotein E; this vaccine was effective in 97% of people ≥ 50 years old 104 .
Recently, TLR agonists have shown excellent potential in activating the immune response. Considering the low expression of some TLRs in elderly individuals, specific TLR agonists may not be appropriate 105 , 106 . Reduced expression of TLR3 and TLR8 in human mDC and TLR7 in pDC leads to reduced cytokine secretion after activation; nevertheless, the expression of TLR1, 2, 4, 5, 6, and 9 did not change with age, and decreased cytokine secretion was also observed, suggesting that other unknown factors besides TLR expression influence the immune response 25 . CpG-ODN can activate TLR9 and promote the proliferation and activation of B cells. Compared to the 3-dose schedule of the hepatitis B vaccine adjuvanted with aluminum, the 2-dose schedule of the vaccine adjuvanted with CpG-ODN resulted in higher serum protection levels in older adults 107 . Lim et al. reported that flagellin-dependent TLR5 expression and signaling are well retained in macrophages from older individuals, similar to that seen in macrophages from young individuals 108 . The pneumococcal surface protein A vaccine adjuvanted with flagellin produced a higher level of specific IgG and IgA responses and showed a high protective effect against Streptococcus pneumoniae in aged mice. Therefore, flagellin-dependent TLR5 is a promising immune adjuvant for older adults. Denton et al. found that targeting TLR4 via its ligand can increase MAdCAM-1 + stromal cell activation and trigger the GC response to immunization 109 . Therefore, age-associated alterations in GC and stromal cell responses can be a target for improving vaccine efficacy in elderly individuals. Other studies also demonstrated that TLR agonist combinations elicited effective immunity in experimental models and may have potential applications in high-efficacy vaccine design for elderly individuals. Zareian et al. reported that the combination of TLR7/TLR8 and TLR4 resulted in higher cytokine secretion by DCs from old adults 110 . This synergistic effect may be due to the combined activation of MyD88 and TRIF-dependent signal transduction pathways.
Useful adjuvants should facilitate innate and adaptive immunity and produce long-lasting protective memory 88 , 111 . Furthermore, adjuvants must strike a balance between reduced inflammation and the low inflammatory state that may hinder vaccine-induced immunity. In view of the underlying chronic inflammation in elderly individuals, adjuvants should improve APC function without eliciting intense inflammatory responses from APC or other immune cells, such as T cells. Ross et al. illustrated that the novel nanoadjuvants (polyanhydride nanoparticles and pentablock copolymer micelles) and cyclic dinucleotides (a STING agonist) have moderate induction of cytokine secretion and weak inflammatory properties, which may be suitable for older adults 112 . In addition, the impacts of adjuvants on vaccine efficacy often result from various mechanisms. Combinations of adjuvants have attracted widespread attention because they can coordinate their respective advantages to maximally enhance immunity 113 . Nanishi et al. found that the TLR9 agonist CpG-ODN can synergize with aluminum hydroxide adjuvant to promote immune protection generated by the SARS-CoV-2 receptor binding domain vaccine in older mice 114 . Future research should focus on adjuvant combinations to induce more comprehensive immune responses.
Inhibiting chronic inflammation
There is increasing research showing that preexisting inflammation can determine vaccine reactivity. Therefore, regulating baseline inflammation before vaccination may be a potential idea to elicit immune responses 49 , 88 . Some studies have attempted to diminish chronic inflammation in older people by administering p38 mitogen-activated protein kinase (MAPK) or mTOR inhibitors before vaccination 115 , 116 . Local rapamycin treatment before vaccination resulted in a 20% increase in antibody titer and a decrease in the number of inhibitory CD4 + and CD8 + T cells 117 . Additionally, as the main source of inflammatory factors, removing senescent cells can also be expected to become an approach to enhance the effectiveness of vaccines 118 . Dasatinib, quercetin, fisetin, and navitoclax were explored as anti-senescent cell therapies that act through different mechanisms 119 , 120 . Moreover, senescent cells upregulated many biomarkers: Senescence-associated β-galactosidase in intracellular lysosomes, which is a classic marker of cellular senescence; CD9 on cell membrane, which regulates many cellular cell adhesion, cell motility, activation, and differentiation; β2-microglobulin, which forms the light chain of MHC-I molecules; and CD47, which functions as a “don’t eat me” signaling molecule 121 . They have shown great potential as targeted delivery ligands for senescent cells.
Metformin is an exciting candidate drug that targets inflammation and inhibits immunosenescence 122 . Metformin was reported to ameliorate Th17 inflammation by inducing autophagy and improving mitochondrial bioenergetics 123 . Other immunomodulators, such as imiquimod and COX-2 inhibitors, were evaluated in terms of their ability to promote vaccine immunity by transiently alleviating chronic inflammation before vaccination 124 , 125 . However, using immunomodulator drugs to target inflammatory signaling pathways has been successfully tested, but the impacts of long-term inhibition and potential side effects are still unclear. Spermidine has pleiotropic properties, including anti-inflammatory, antioxidant, and molecular chaperone activity; furthermore, spermidine enhances mitochondrial metabolism and modulates protein homeostasis 126 . Many of the anti-aging effects of spermidine are related to its polyamine-induced cytoprotection of autophagy. Spermidine was reported to regulate macrophage differentiation and alleviate colitis symptoms in mice 127 . Epigallocatechin gallate (EGCG) is a natural product extracted from green tea that contains a phenolic structure with three hydroxyl groups with various activities, such as anti-inflammatory, antioxidant, antitumor, and antibacterial activities 128 . Recent studies have found that EGCG can reduce aging-associated NF-κB inflammation and oxidative stress, thereby prolonging the life of healthy rats 129 , 130 . Interestingly, EGCG also has adjuvant effects when co-administered with influenza virus HA antigen, which induces a large number of neutralizing antibodies 131 . Incorporating these substances with both anti-inflammatory and adjuvant effects into vaccine formulations will be a potential strategy for future vaccine design.
In addition to drugs that target senescent cells and inhibit inflammation, finding target cells that efficiently inhibit inflammation pathways is another strategy. Vaccine-induced ICOS + CD38 + circulating Tfh cells from elderly individuals stimulate TNF-NF-κB inflammatory pathway activation 132 . Studies suggest that ICOS + CD38 + circulating Tfh cells are essential for the design of vaccines that target age-related alterations to the inflammation pathway.
Inhibiting immunosenescence
Currently, the main measures to elevate vaccination efficiency in elderly individuals include increasing the antigen dose, preparing multivalent vaccines, performing multiple immunizations, and adding adjuvants to boost the immune response. These approaches aim to optimize the vaccine delivery system but often ignore the influence of the immune microenvironment of older individuals on the immune response. Without fundamentally improving the immunosenescence of elderly individuals, vaccines will not be able to produce strong and long-lasting immune efficacy. Inhibiting immunosenescence in elderly individuals will be a critical measure to improve immune responses to vaccines. Finding adjuvants and vaccine components that can inhibit immunosenescence is an attractive direction for future research.
An increasing number of studies have shown that T cell aging is the major reason for diseases in elderly individuals 133 . T cells are also vital effector cells for vaccines, exert cellular immunity, and kill infected or tumor cells 134 , 135 . The ability of T cells to recognize and eliminate pathogenic microorganisms or tumor cells significantly decreases with age 136 . In particular, the immunosenescence of CD8 + T cells in tumor-draining lymph nodes enables tumor cells to escape immune surveillance and elimination and promotes tumor progression 137 . Senescent T cells in the tumor microenvironment have become a new target for tumor immunotherapy 138 . However, nanovaccines to reverse senescent T cells have not yet been reported.
Most studies have reported that improving immunity in older people involves inhibiting T cell senescence. Interactions between APCs and T cells determine the intensity of the immune response 139 . Therefore, restoring the function of APCs and T cells and their relationship is pivotal to improving the immune efficiency of elderly individuals. Determining how to simultaneously alleviate the immunosenescence of APCs and T cells to facilitate vaccine protection will be the focus.
Notably, natural killer (NK) cells are an important part of innate immunity and play an irreplaceable role in maintaining health, anti-infection, and antitumor functions in elderly individuals. Studies have shown that NK cells can recognize and clear senescent cells in a variety of ways, such as direct killing and the secretion of cytokines or perforins 140 . These findings imply that increasing the amount and improving the function of NK cells may be another option to promote the efficiency of vaccines based on anti-aging strategies.
Current design strategies and examples
The current design strategies and examples of vaccines for elderly individuals are shown in Fig. 4 and Table 1 . The differences in the effectiveness of the vaccine in older people also depend on whether it is a primary or booster vaccination. Primary vaccination with the herpes zoster subunit vaccine and the COVID-19 mRNA vaccine also resulted in high efficacy in the elderly population. Recombinant subunit herpes zoster vaccine can provide effective immune protection due to the adjuvant AS01, which is essential for improving immunity in the elderly. COVID-19 mRNA vaccines were approved for emergency use during the COVID-19 pandemic 141 . The mechanism may be that mRNA can be recognized by TLR3, 7, and 8 to induce adjuvant-like activity and enhanced antigen expression and antigen presentation 8 . Therefore, there is a strong interest in using mRNA vaccines against other diseases in the elderly. Moreover, booster vaccination may offset the decline in vaccine responses in older adults. When older adults receive two doses of BNT162b2 vaccine three weeks apart, the neutralizing antibodies of SARS-CoV-2 weaken over time, but a third booster dose of the vaccine can further increase antibody levels and cross-protection against Omicron BA.1 and BA.2 142 . Meanwhile, the interval between the two doses may also influence the effectiveness of the vaccines. In a population-based cohort study, participants were boosted with the BNT162b2 vaccine at three weeks or longer intervals (11–12 weeks). The results showed that the peak antibody response was 3.5-fold higher in participants who received the delayed interval vaccination 143 .
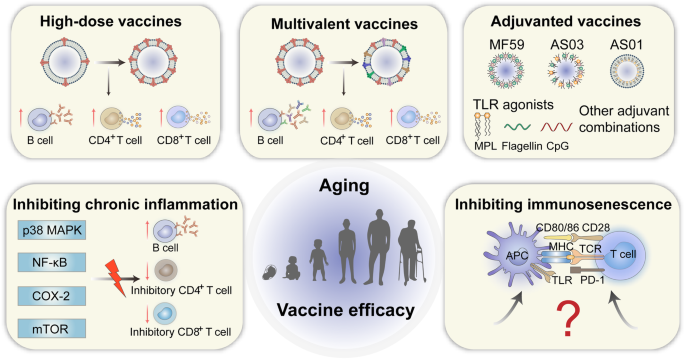
AS adjuvant system, MPL monophosphoryl lipid, TLR toll-like receptor, MAPK mitogen-activated protein kinase, NF-κB nuclear factor kappa-light-chain-enhancer of activated B cells; COX cyclooxygenase; mTOR mammalian target of rapamycin; APC antigen-presenting cell; MHC major histocompatibility complex; TCR T cell receptor; PD-1 programmed death receptor-1. (Created with Adobe illustrator).
Conclusions and perspectives
The global population is entering an era of aging. Older people are more susceptible to pathogens and have higher rates of morbidity and mortality 144 . Despite the significant success of current vaccine products, many commercial vaccines fail to generate effective and long-lasting immune protection in elderly individuals. With increasing age, the reasons for the decline in vaccine potency are multifactorial. Age-related dysregulation of lymph nodes, and crucial immune cells jointly reduces the efficiency of vaccination. With the continuous emergence of new pathogens, it is urgent to create strategies to improve vaccination-mediated protection for elderly individuals.
This review summarized a series of approaches to provide a reference for vaccine design for elderly individuals. We first analyzed the characteristics of immunosenescence in the elderly population and outlined the impacts of these changes on the potency of vaccination. The main measures to enhance vaccination efficiency in the elderly are increasing the antigen dose, preparing multivalent vaccines, multiple immunizations, and adding adjuvants to enhance immune responses. Subsequently, we discussed current strategies for improving immune responses to vaccines, analyzed their advantages and limitations, and proposed potential directions for future research.
The existing approaches are primarily aimed at optimizing the vaccine delivery system rather than inhibiting the immunosenescence of the immune microenvironment in elderly individuals. Inhibiting the immunosenescence of elderly individuals can evoke strong and long-lasting immune protection, which serves as a critical measure to improve vaccine-induced immunity. Although inhibition of immunosenescence most likely requires continuous intervention/treatment and is complicated to achieve, we believe that sustained-release vaccination/adjuvants or booster immunizations may sustainably ameliorate immunosenescence in the elderly. Once the immunosenescence of the elderly is corrected, their immune efficacy against various antigens can be improved. An attractive research direction will be discovering immunomodulators and vaccine formulations that can inhibit immunosenescence. The selection of adjuvants can greatly impact the type and magnitude of the immune response 145 . Considering the special immune status of elderly individuals, designing tailored vaccine adjuvants is indispensable for the development of next-generation vaccines for older individuals. A chronic inflammatory state also accompanies immunosenescence. However, the common opinion is that adjuvants promote immunity by inducing local inflammation. Therefore, more in-depth studies are needed to explain the role of inflammation in vaccine-induced immunity and tune the contradictory perspectives.
In addition to the described methods to improve vaccination efficiency, there are also studies to alter the route of administration. Vaccines are generally injected subcutaneously and intramuscularly. Some studies have explored the efficacy of vaccines administered via other routes. Clinical studies have indicated that intradermally injecting influenza vaccine can improve the titer and seroconversion rates in elderly individuals after vaccination 146 . The dermis contains many specialized DCs, such as LCs, which can present antigens 26 . However, an intradermal injection can cause more pain and local adverse reactions. The mucosal routes, including oral, intranasal, and vaginal vaccination, may be promising approaches. A study comparing intranasal and intramuscular vaccination in older populations showed that seroconversion rates are similar, but intranasal vaccination can generate more mucosal IgA responses 147 . For efficient nasal delivery, vaccines should be internalized by microfold cells and DCs in nasal-associated lymphoid tissues 148 . In addition, vaccine design needs to account for more factors, such as the limited volume of administration and the movement of nasal cilia leading to vaccine clearance. Thus, it is necessary to study alternative vaccination routes for elderly individuals further.
During clinical research, observing different immune outcomes in animal models and human settings is common. The characteristics and functional differences in anatomical structures, disease models, and TLR expression profiles between humans and mice have pivotal impacts. Additionally, many aging-related experiments in elderly mice were performed under specific pathogen-free conditions, which have some limitations. Older individuals may be in a frail state with multiple underlying diseases and comorbidities, which may also affect vaccine potency 18 , 149 . The ongoing evolution and immune imprinting of influenza viruses and SARS-CoV-2 strains is an important impediment to the effectiveness of vaccines against new variants 150 . Once the immune system has built up an immune memory for an antigen, when it encounters a new, similar antigen, it will preferentially activate the immune response against the previous antigen. It is desirable if a response to a shared epitope results in virus neutralization, but it can be detrimental if a response to a non-protective epitope is dominant. In aged mice, immune imprinting of influenza virus reduced the efficacy of the vaccine against Streptococcus pneumoniae 151 . Wang et al. developed a method that measures the antigenic distance between different strains to predict the immune response to a particular strain 152 . Animal studies suggest that using dendritic cell-activating adjuvants and repeated immunization may overcome immune imprinting 153 , and these measures can also improve the efficacy of the vaccine in the elderly. We believe that the ideal vaccine response would overcome immune imprinting and combat a broader spectrum of pathogens and variants. Such universal vaccines could prevent the virus from mutating to the point where it escapes the immune system and could ultimately be the key to controlling future pandemics.
Most studies focus on one or several cell types or certain processes of the immune response. However, our immune system is a complex and coordinated comprehensive network. More new technologies and advances will help reveal the complexity underlying the human immune system. We must pay more attention to the impacts of versatile cells or multiple immune cascade processes. Future research should focus on developing scientific methods to build more convincing models of aging and study the profound mechanisms underlying age-related alterations that impact the immune responses of older people. Additionally, interdisciplinary research involving vaccinology, immunology, and artificial intelligence may also assist in the research and development of vaccines; this collaboration could provide more innovative strategies and spark ideas for personalized vaccine design.
Data availability
Data availability is not applicable to this article as no new data were created or analyzed in this study.
Li, X. et al. Inflammation and aging: signaling pathways and intervention therapies. Signal Transduct. Target Ther. 8 , 239 (2023).
Article PubMed PubMed Central Google Scholar
United Nations. Department of Economic and Social Affairs, Population Division (2019). World Population Ageing 2019: Highlights (ST/ESA/SER.A/430) (2022).
Gralinski, L. E. & Menachery, V. D. Return of the Coronavirus: 2019-nCoV. Viruses 12 , 135 (2020).
Koff, W. C. et al. Accelerating next-generation vaccine development for global disease prevention. Science 340 , 1232910 (2013).
Mascola, J. R. & Fauci, A. S. Novel vaccine technologies for the 21st century. Nat. Rev. Immunol. 20 , 87–88 (2020).
Article CAS PubMed Google Scholar
Hou, Y. et al. Advanced subunit vaccine delivery technologies: from vaccine cascade obstacles to design strategies. Acta Pharm. Sin. B 13 , 3321–3338 (2023).
Article CAS PubMed PubMed Central Google Scholar
Cunningham, A. L., McIntyre, P., Subbarao, K., Booy, R. & Levin, M. J. Vaccines for older adults. BMJ 372 , n188 (2021).
Article PubMed Google Scholar
Bell, M. R. & Kutzler, M. A. An old problem with new solutions: strategies to improve vaccine efficacy in the elderly. Adv. Drug Deliv. Rev. 183 , 114175 (2022).
Osterholm, M. T. Efficacy and effectiveness of influenza vaccines: a systematic review and meta-analysis. Lancet Infect. Dis. 12 , 36–44 (2012).
Walford, R. L. The immunologic theory of aging. Gerontologist 4 , 195–197 (1964).
Willyard, C. How anti-ageing drugs could boost COVID vaccines in older people. Nature 586 , 352–354 (2020).
Qin, X., Jian, D. & Yi, C. Role of CD8 + T lymphocyte cells: interplay with stromal cells in tumor microenvironment. Acta Pharm. Sin. B 11 , 1365–1378 (2021).
Article Google Scholar
Riese, P. et al. Distinct immunological and molecular signatures underpinning influenza vaccine responsiveness in the elderly. Nat. Commun. 13 , 6894 (2022).
Roukens, A. H. et al. Elderly subjects have a delayed antibody response and prolonged viraemia following yellow fever vaccination: a prospective controlled cohort study. PloS One 6 , e27753 (2011).
Schulz, A. R. et al. Low thymic activity and dendritic cell numbers are associated with the immune response to primary viral infection in elderly humans. J. Immunol. 195 , 4699–4711 (2015).
Ding, Y., Li, Z., Jaklenec, A. & Hu, Q. Vaccine delivery systems toward lymph nodes. Adv. Drug Deliv. Rev. 179 , 113914 (2021).
Lefebvre, J. S., Masters, A. R., Hopkins, J. W. & Haynes, L. Age-related impairment of humoral response to influenza is associated with changes in antigen specific T follicular helper cell responses. Sci. Rep. 6 , 25051 (2016).
Chen, J., Deng, J. C. & Goldstein, D. R. How aging impacts vaccine efficacy: known molecular and cellular mechanisms and future directions. Trends Mol. Med. 28 , 1100–1111 (2022).
Hadamitzky, C. et al. Age-dependent histoarchitectural changes in human lymph nodes: an underestimated process with clinical relevance? J. Anat. 216 , 556–562 (2010).
Agrawal, A. et al. Altered innate immune functioning of dendritic cells in elderly humans: a role of phosphoinositide 3-kinase-signaling pathway. J. Immunol. 178 , 6912–6922 (2007).
Yang, Y., Guo, X., Hu, B., He, P. & Feng, M. Generated SecPen_NY-ESO-1_ubiquitin-pulsed dendritic cell cancer vaccine elicits stronger and specific T cell immune responses. Acta Pharm. Sin. B 11 , 476–487 (2020).
Eisenbarth, S. C. Dendritic cell subsets in T cell programming: location dictates function. Nat. Rev. Immunol. 19 , 89–103 (2019).
Heath, W. R., Kato, Y., Steiner, T. M. & Caminschi, I. Antigen presentation by dendritic cells for B cell activation. Curr. Opin. Immunol. 58 , 44–52 (2019).
Wang, J., Geiger, H. & Rudolph, K. L. Immunoaging induced by hematopoietic stem cell aging. Curr. Opin. Immunol. 23 , 532–536 (2011).
Panda, A. et al. Age-associated decrease in TLR function in primary human dendritic cells predicts influenza vaccine response. J. Immunol. 184 , 2518–2527 (2010).
Leleux, J., Atalis, A. & Roy, K. Engineering immunity: modulating dendritic cell subsets and lymph node response to direct immune-polarization and vaccine efficacy. J. Control. Release 219 , 610–621 (2015).
Jackaman, C. et al. Targeting macrophages rescues age-related immune deficiencies in C57BL/6J geriatric mice. Aging Cell 12 , 345–357 (2013).
Prieto, L. I. et al. Senescent alveolar macrophages promote early-stage lung tumorigenesis. Cancer Cell 41 , 1261–1275.e6 (2023).
Wang, J., Yang, J. & Kopecek, J. Nanomedicines in B cell-targeting therapies. Acta Biomater. 137 , 1–19 (2022).
Frasca, D. & Blomberg, B. B. Aging affects human B cell responses. J. Clin. Immunol. 31 , 430–435 (2011).
Pritz, T. et al. Plasma cell numbers decrease in bone marrow of old patients. Eur. J. Immunol. 45 , 738–746 (2015).
Cancro, M. P. Age-associated B cells. Annu. Rev. Immunol. 38 , 315–340 (2020).
Yam-Puc, J. C. et al. Age-associated B cells predict impaired humoral immunity after COVID-19 vaccination in patients receiving immune checkpoint blockade. Nat. Commun. 14 , 3292 (2023).
Allen, C. D. et al. Germinal center dark and light zone organization is mediated by CXCR4 and CXCR5. Nat. Immunol. 5 , 943–952 (2004).
Wols, H. A. et al. Migration of immature and mature B cells in the aged microenvironment. Immunology 129 , 278–290 (2010).
Frasca, D., Blomberg, B. B., Garcia, D., Keilich, S. R. & Haynes, L. Age-related factors that affect B cell responses to vaccination in mice and humans. Immunol. Rev. 296 , 142–154 (2020).
Lefebvre, J. S. et al. The aged microenvironment contributes to the age‐related functional defects of CD4 T cells in mice. Aging Cell 11 , 732–740 (2012).
Silva-Cayetano, A. et al. Spatial dysregulation of T follicular helper cells impairs vaccine responses in aging. Nat. Immunol. 24 , 1124–1137 (2023).
Khurana, S., Frasca, D., Blomberg, B. & Golding, H. AID activity in B cells strongly correlates with polyclonal antibody affinity maturation in-vivo following pandemic 2009-H1N1 vaccination in humans. PLoS Pathog. 8 , e1002920 (2012).
Stiasny, K., Aberle, J. H., Keller, M., Grubeck-Loebenstein, B. & Heinz, F. X. Age affects quantity but not quality of antibody responses after vaccination with an inactivated flavivirus vaccine against tick-borne encephalitis. PLoS One 7 , e34145 (2012).
Goronzy, J. J. & Weyand, C. M. Understanding immunosenescence to improve responses to vaccines. Nat. Immunol. 14 , 428–436 (2013).
Chen, X., Liu, Q. & Xiang, A. P. CD8 + CD28 - T cells: not only age-related cells but a subset of regulatory T cells. Cell Mol. Immunol. 15 , 734–736 (2018).
Kumagai, S. et al. The PD-1 expression balance between effector and regulatory T cells predicts the clinical efficacy of PD-1 blockade therapies. Nat. Immunol. 21 , 1346–1358 (2020).
Gustafson, C. E., Weyand, C. M. & Goronzy, J. J. T follicular helper cell development and functionality in immune ageing. Clin. Sci. 132 , 1925–1935 (2018).
Article CAS Google Scholar
Herati, R. S. et al. Circulating CXCR5 + PD-1 + response predicts influenza vaccine antibody responses in young adults but not elderly adults. J. Immunol. 193 , 3528–3537 (2014).
Franceschi, C. et al. Inflamm aging: an evolutionary perspective on immunosenescence. Ann. N.Y. Acad. Sci. 908 , 244–254 (2000).
Franceschi, C. et al. Inflammaging and ‘Garb-aging. Trends Endocrinol. Metab. 28 , 199–212 (2017).
Gilroy, D. & De Maeyer, R. New insights into the resolution of inflammation. Semin. Immunol. 27 , 161–168 (2015).
Chambers, E. S. & Akbar, A. N. Can blocking inflammation enhance immunity during aging? J. Allergy Clin. Immunol. 145 , 1323–1331 (2020).
Hadrup, S. R. et al. Longitudinal studies of clonally expanded CD8 T cells reveal a repertoire shrinkage predicting mortality and an increased number of dysfunctional cytomegalovirus-specific T cells in the very elderly. J. Immunol. 176 , 2645–2653 (2006).
Frasca, D., Blomberg, B. B. & Paganelli, R. Aging, obesity, and inflammatory age-related diseases. Front. Immunol. 8 , 1745 (2017).
Kim, K. A., Jeong, J. J., Yoo, S. Y. & Kim, D. H. Gut microbiota lipopolysaccharide accelerates inflamm-aging in mice. BMC Microbiol 16 , 9 (2016).
Claesson, M. J. et al. Gut microbiota composition correlates with diet and health in the elderly. Nature 488 , 178–184 (2012).
De Maeyer, R. P. H. et al. Blocking elevated p38 MAPK restores efferocytosis and inflammatory resolution in the elderly. Nat. Immunol. 21 , 615–625 (2020).
Lasry, A. & Ben-Neriah, Y. Senescence-associated inflammatory responses: aging and cancer perspectives. Trends Immunol. 36 , 217–228 (2015).
Gulen, M. F. et al. cGAS-STING drives ageing-related inflammation and neurodegeneration. Nature 620 , 374–380 (2023).
Gritsenko, A., Green, J. P., Brough, D. & Lopez-Castejon, G. Mechanisms of NLRP3 priming in inflammaging and age-related diseases. Cytokine Growth Factor Rev. 55 , 15–25 (2020).
Lim, S.O. et al. Deubiquitination and stabilization of PD-L1 by CSN5. Cancer Cell , 925–939 (2016).
Hamilton, J. A. G. et al. Interleukin-37 improves T-cell-mediated immunity and chimeric antigen receptor T-cell therapy in aged backgrounds. Aging Cell 20 , e13309 (2021).
Chen, X., Baumel, M., Männel, D. N., Howard, O. M. Z. & Oppenheim, J. J. Interaction of TNF with TNF receptor type 2 promotes expansion and function of mouse CD4 + CD25 + T regulatory cells. J. Immunol. 179 , 154–161 (2007).
Watanabe, R., Shirai, T., Hong, N., Zhang, H. & Weyand, C. M. Pyruvate controls the checkpoint inhibitor PD-L1 and suppresses T cell immunity. J. Clin. Investig. 127 , 2725–2738 (2017).
Chen, J. H. et al. Prostaglandin E2 and programmed cell death 1 signaling coordinately impair CTL function and survival during chronic viral infection. Nat. Med. 21 , 327–334 (2015).
López-Otín, C., Blasco, M. A., Partridge, L., Serrano, M. & Kroemer, G. Hallmarks of aging: an expanding universe. Cell 186 , 243–278 (2023).
Guo, J. et al. Aging and aging-related diseases: from molecular mechanisms to interventions and treatments. Signal Transduct. Target Ther. 7 , 391 (2022).
Hansen, M., Rubinsztein, D. C. & Walker, D. W. Autophagy as a promoter of longevity: insights from model organisms. Nat. Rev. Mol. Cell Biol. 19 , 579–593 (2018).
Zhang, H. et al. Polyamines control eIF5A hypusination, TFEB translation, and autophagy to reverse B cell senescence. Mol. Cell. 76 , 110–125.e9 (2019).
Aman, Y. et al. Autophagy in healthy aging and disease. Nat. Aging 1 , 634–650 (2021).
Kim, J., Kundu, M., Viollet, B. & Guan, K. L. AMPK and mTOR regulate autophagy through direct phosphorylation of Ulk1. Nat. Cell Biol. 13 , 132–141 (2011).
Cirone, M. Perturbation of bulk and selective macroautophagy, abnormal UPR activation and their interplay pave the way to immune dysfunction, cancerogenesis and neurodegeneration in ageing. Ageing Res. Rev. 58 , 101026 (2020).
Wang, Y. et al. In situ manipulation of dendritic cells by an autophagy-regulative nanoactivator enables effective cancer immunotherapy. ACS Nano 13 , 7568–7577 (2019).
Wang, S. et al. Exploration of antigen-induced CaCO 3 nanoparticles for therapeutic vaccine. Small 14 , e1704272 (2018).
Hubbard, V. M. et al. Macroautophagy regulates energy metabolism during effector T cell activation. J. Immunol. 185 , 7349–7357 (2010).
Fan, J., Feng, Z. & Chen, N. Spermidine as a target for cancer therapy. Pharmacol. Res. 159 , 104943 (2020).
De Risi, M. et al. Mechanisms by which autophagy regulates memory capacity in ageing. Aging Cell 19 , e13189 (2020).
Ma, T. et al. Low-dose metformin targets the lysosomal AMPK pathway through PEN2. Nature 603 , 159–165 (2022).
Kroemer, G. & Zitvogel, L. CD4 + T cells at the center of inflammaging. Cell Metab. 32 , 4–5 (2020).
Chakravarti, D., LaBella, K. A. & DePinho, R. A. Telomeres: history, health, and hallmarks of aging. Cell 184 , 306–322 (2021).
Lanna, A. et al. An intercellular transfer of telomeres rescues T cells from senescence and promotes long-term immunological memory. Nat. Cell Biol. 24 , 1461–1474 (2022).
Gravenstein, S. et al. Comparative effectiveness of high-dose versus standard-dose influenza vaccination on numbers of US nursing home residents admitted to hospital: a cluster-randomised trial. Lancet Respir. Med. 5 , 738–746 (2017).
Couch, R. B. et al. Safety and immunogenicity of a high dosage trivalent influenza vaccine among elderly subjects. Vaccine 25 , 7656–7663 (2007).
Wilkinson, K. et al. Efficacy and safety of high-dose influenza vaccine in elderly adults: a systematic review and meta-analysis. Vaccine 35 , 2775–2780 (2017).
Goronzy, J. J., Fang, F., Cavanagh, M. M., Qi, Q. & Weyand, C. M. Naive T cell maintenance and function in human aging. J. Immunol. 194 , 4073–4080 (2015).
Sun, Y. et al. Metal-organic framework nanocarriers for drug delivery in biomedical applications. Nanomicro Lett. 12 , 103 (2020).
CAS PubMed PubMed Central Google Scholar
Hong, X. et al. The pore size of mesoporous silica nanoparticles regulates their antigen delivery efficiency. Sci. Adv. 6 , eaaz4462 (2020).
Xia, Y. et al. Exploiting the pliability and lateral mobility of Pickering emulsion for enhanced vaccination. Nat. Mater. 17 , 187–194 (2018).
Liu, K. et al. A novel multifunctional vaccine platform with dendritic cell-targeting and pH-responsive for cancer immunotherapy: antigen-directed biomimetic fabrication of a cabbage-like mannatide-zinc-antigen hybrid microparticles. Chem. Eng. J. 426 , 130867 (2021).
Zhao, J. et al. A minimalist binary vaccine carrier for personalized postoperative cancer vaccine therapy. Adv. Mater. 34 , e2109254 (2022).
Pereira, B., Xu, X. N. & Akbar, A. N. Targeting inflammation and immunosenescence to improve vaccine responses in the rlderly. Front. Immunol. 11 , 583019 (2020).
Lauer, K. B., Borrow, R. & Blancharda, T. J. Multivalent and multipathogen viral vector vaccines. Clin. Vaccin. Immunol. 24 , e00298–16 (2017).
Lewnard, J. A. et al. Effectiveness of 13-Valent pneumococcal conjugate vaccine against medically attended lower respiratory tract infection and pneumonia among older adults. Clin. Infect. Dis. 75 , 832–841 (2022).
Lawrence, H. et al. Effectiveness of the 23-valent pneumococcal polysaccharide vaccine against vaccine serotype pneumococcal pneumonia in adults: a case-control test-negative design study. PLoS Med. 17 , e1003326 (2020).
Hernandez-Davies, J. E. et al. Administration of multivalent influenza virus recombinant hemagglutinin vaccine in combination-adjuvant elicits broad reactivity beyond the vaccine components. Front. Immunol. 12 , 692151 (2021).
Reed, S. G., Orr, M. T. & Fox, C. B. Key roles of adjuvants in modern vaccines. Nat. Med. 19 , 1597–1608 (2013).
Pulendran, B., Arunachalam, P. S. & O’Hagan, D. T. Emerging concepts in the science of vaccine adjuvants. Nat. Rev. Drug Discov. 20 , 454–475 (2021).
Peletta, A., Lemoine, C., Courant, T., Collin, N. & Borchard, G. Meeting vaccine formulation challenges in an emergency setting: towards the development of accessible vaccines. Pharmacol. Res. 189 , 106699 (2023).
Nanishi, E. et al. Precision vaccine adjuvants for older adults: a scoping review. Clin. Infect. Dis. 75 , S72–S80 (2022).
Ciabattini, A. et al. Vaccination in the elderly: the challenge of immune changes with aging. Semin. Immunol. 40 , 83–94 (2018).
Nicolay, U., Heijnen, E., Nacci, P., Patriarca, P. A. & Leav, B. Immunogenicity of aIIV3, MF59-adjuvanted seasonal trivalent influenza vaccine, in older adults ≥65 years of age: meta-analysis of cumulative clinical experience. Int. J. Infect. Dis. 85S , S1–S9 (2019).
Isakova Sivak, I. & Rudenko, L. Cross-protective potential of a MF59-adjuvanted quadrivalent influenza vaccine in older adults. Lancet Infect. Dis. 21 , 900–901 (2021).
Morel, S. et al. Adjuvant System AS03 containing α-tocopherol modulates innate immune response and leads to improved adaptive immunity. Vaccine 29 , 2461–2473 (2011).
Yam, K. K. et al. AS03-adjuvanted, very-low-dose influenza vaccines induce distinctive immune responses compared to unadjuvanted high-dose vaccines in BALB/c mice. Front. Immunol. 6 , 207 (2015).
Dendouga, N., Fochesato, M., Lockman, L., Mossman, S. & Giannini, S. L. Cell-mediated immune responses to a varicella-zoster virus glycoprotein E vaccine using both a TLR agonist and QS21 in mice. Vaccine 30 , 3126–3135 (2012).
Nam, H. J. et al. An adjuvanted zoster vaccine elicits potent cellular immune responses in mice without QS21. NPJ Vaccines 7 , 45 (2022).
Lal, H. et al. Efficacy of an adjuvanted herpes zoster subunit vaccine in older adults. N. Engl. J. Med. 372 , 2087–2096 (2015).
Renshaw, M. et al. Cutting Edge: impaired toll-like receptor expression and function in aging. J. Immunol. 169 , 4697–4701 (2002).
Metcalf, T. U. et al. Global analyses revealed age-related alterations in innate immune responses after stimulation of pathogen recognition receptors. Aging Cell 14 , 421–432 (2015).
Janssen, J. M., Jackson, S., Heyward, W. L. & Janssen, R. S. Immunogenicity of an investigational hepatitis B vaccine with a toll-like receptor 9 agonist adjuvant (HBsAg-1018) compared with a licensed hepatitis B vaccine in subpopulations of healthy adults 18-70 years of age. Vaccine 33 , 3614–3618 (2015).
Lim, J. S. et al. Flagellin-dependent TLR5/caveolin-1 as a promising immune activator in immunosenescence. Aging Cell 14 , 907–915 (2015).
Denton, A. E. et al. Targeting TLR4 during vaccination boosts MAdCAM-1 + lymphoid stromal cell activation and promotes the aged germinal center response. Sci. Immunol. 7 , eabk0018 (2022).
Zareian, N. et al. Triggering of toll-like receptors in old individuals. Relevance for vaccination. Curr. Pharm. Des. 25 , 4163–4167 (2019).
Wu, T. Y. et al. Rational design of small molecules as vaccine adjuvants. Sci. Transl. Med. 6 , 263ra160 (2014).
Ross, K. A. et al. Novel nanoadjuvants balance immune activation with modest inflammation: implications for older adult vaccines. Immun. Ageing 20 , 28 (2023).
Ananya, A. et al. Just right” combinations of adjuvants with nanoscale carriers activate aged dendritic cells without overt inflammation. Immun. Ageing 20 , 10 (2023).
Nanishi, E., Borriello, F., O’Meara, T. R., Mcgrath, M. E. & Dowling, D. J. An aluminum hydroxide:CpG adjuvant enhances protection elicited by a SARS-CoV-2 receptor-binding domain vaccine in aged mice. Sci. Transl. Med. 14 , eabj5305 (2021).
Lanna, A. et al. A sestrin-dependent Erk-Jnk-p38 MAPK activation complex inhibits immunity during aging. Nat. Immunol. 18 , 354–363 (2017).
Kennedy, R. B. et al. Immunosenescence-related transcriptomic and immunologic changes in older individuals following influenza vaccination. Front. Immunol. 7 , 450 (2016).
Mannick, J. B. et al. mTOR inhibition improves immune function in the elderly. Sci. Transl. Med. 6 , 268ra179 (2014).
Song, S., Lam, E. W. F., Tchkonia, T., Kirkland, J. L. & Sun, Y. Senescent cells: emerging targets for human aging and age-related diseases. Trends Biochem. Sci. 45 , 578–592 (2020).
Wissler Gerdes, E. O., Misra, A., Netto, J. M. E., Tchkonia, T. & Kirkland, J. L. Strategies for late phase preclinical and early clinical trials of senolytics. Mech. Ageing Dev. 200 , 111591 (2021).
Kirkland, J. L. & Tchkonia, T. Senolytic drugs: from discovery to translation. J. Intern. Med. 288 , 518–536 (2020).
Zhang, Q., Li, S., Chen, F., Zeng, R. & Tong, R. Targeted delivery strategy: a beneficial partner for emerging senotherapy. Biomed. Pharmacother. 155 , 113737 (2022).
Chen, S. et al. Metformin in aging and aging-related diseases: clinical applications and relevant mechanisms. Theranostics 12 , 2722–2740 (2022).
Bharath, L. P. et al. Metformin enhances autophagy and normalizes mitochondrial function to alleviate aging-associated inflammation. Cell Metab. 32 , 44–55.e6 (2020).
Hung, I. F. et al. Immunogenicity of intradermal trivalent influenza vaccine with topical imiquimod: a double blind randomized controlled trial. Clin. Infect. Dis. 59 , 1246–1255 (2014).
Pettersen, F. O. et al. An exploratory trial of cyclooxygenase type 2 inhibitor in HIV-1 infection: downregulated immune activation and improved T cell-dependent vaccine responses. J. Virol. 85 , 6557–6566 (2011).
Madeo, F., Eisenberg, T., Pietrocola, F. & Kroemer, G. Spermidine in health and disease. Science 359 , eaan2788 (2018).
Nakamura, A. et al. Symbiotic polyamine metabolism regulates epithelial proliferation and macrophage differentiation in the colon. Nat. Commun. 12 , 2105 (2021).
Aggarwal, V. et al. Molecular mechanisms of action of epigallocatechin gallate in cancer: recent trends and advancement. Semin. Cancer Biol. 80 , 256–275 (2022).
Yuan, H. et al. The phytochemical epigallocatechin gallate prolongs the lifespan by improving lipid metabolism, reducing inflammation and oxidative stress in high-fat diet-fed obese rats. Aging Cell 19 , e13199 (2020).
Tavenier, J. et al. Alterations of monocyte NF-kappaB p65/RelA signaling in a cohort of older medical patients, age-matched controls, and healthy young adults. Immun. Ageing 17 , 25 (2020).
Cheong, Y. et al. Epigallocatechin-3-Gallate as a novel vaccine adjuvant. Front. Immunol. 12 , 769088 (2021).
Herati, R. S. et al. Vaccine-induced ICOS + CD38 + circulating Tfh are sensitive biosensors of age-related changes in inflammatory pathways. Cell Rep. Med. 2 , 100262 (2021).
Carrasco, E. et al. The role of T cells in age-related diseases. Nat. Rev. Immunol. 22 , 97–111 (2022).
Heitmann, J. S. et al. A COVID-19 peptide vaccine for the induction of SARS-CoV-2 T cell immunity. Nature 601 , 617–622 (2022).
Hu, Y. et al. Synergistic tumor immunological strategy by combining tumor nanovaccine with gene-mediated extracellular matrix scavenger. Biomaterials 252 , 120114 (2020).
Mittelbrunn, M. & Kroemer, G. Hallmarks of T cell aging. Nat. Immunol. 22 , 687–698 (2021).
Fear, V. S. et al. Tumour draining lymph node-generated CD8 T cells play a role in controlling lung metastases after a primary tumour is removed but not when adjuvant immunotherapy is used. Cancer Immunol. Immunother. 70 , 3259 (2021).
Liu, X., Hoft, D. F. & Peng, G. Senescent T cells within suppressive tumor microenvironments: emerging target for tumor immunotherapy. J. Clin. Investig. 130 , 1073–1083 (2020).
Liu, C. et al. A nanovaccine for antigen self-presentation and immunosuppression reversal as a personalized cancer immunotherapy strategy. Nat. Nanotechnol. 17 , 531–540 (2022).
Antonangeli, F., Zingoni, A., Soriani, A. & Santoni, A. Senescent cells: living or dying is a matter of NK cells. J. Leukoc. Biol. 105 , 1275–1283 (2019).
Chaudhary, N., Weissman, D. & Whitehead, K. A. mRNA vaccines for infectious diseases: principles, delivery and clinical translation. Nat. Rev. Drug Discov. 20 , 817–838 (2021).
Newman, J. et al. Neutralizing antibody activity against 21 SARS-CoV-2 variants in older adults vaccinated with BNT162b2. Nat. Microbiol. 7 , 1180–1188 (2022).
Parry, H. et al. Extended interval BNT162b2 vaccination enhances peak antibody generation. NPJ Vaccines 7 , 14 (2022).
Thompson, W. W. et al. Mortality associated with influenza and respiratory syncytial virus in the United States. JAMA 289 , 179–186 (2003).
Di Pasquale, A., Preiss, S., Tavares Da Silva, F. & Garcon, N. Vaccine adjuvants: from 1920 to 2015 and beyond. Vaccines 3 , 320–343 (2015).
Tsang, P. et al. Immunogenicity and safety of Fluzone® intradermal and high-dose influenza vaccines in older adults ≥65 years of age: a randomized, controlled, phase II trial. Vaccine 32 , 2507–2517 (2014).
Muszkat, M. et al. Local and systemic immune response in nursing-home elderly following intranasal or intramuscular immunization with inactivated influenza vaccine. Vaccine 21 , 1180–1186 (2003).
Mosafer, J., Sabbaghi, A. H., Badiee, A., Dehghan, S. & Tafaghodi, M. Preparation, characterization and in vivo evaluation of alginate-coated chitosan and trimethylchitosan nanoparticles loaded with PR8 influenza virus for nasal immunization. Asian J. Pharm. Sci. 14 , 216–221 (2019).
Andrew, M. K. et al. The importance of frailty in the assessment of influenza vaccine effectiveness against influenza-related hospitalization in elderly people. J. Infect. Dis. 216 , 405–414 (2017).
Huang, C. Q., Vishwanath, S., Carnell, G. W., Chan, A. C. Y. & Heeney, J. L. Immune imprinting and next-generation coronavirus vaccines. Nat. Microbiol. 8 , 1971–1985 (2023).
Jirru, E. et al. Impact of influenza on pneumococcal vaccine effectiveness during Streptococcus pneumoniae infection in aged murine lung. Vaccines 8 , 298 (2020).
Wang, J. et al. Broadly reactive IgG responses to heterologous H5 prime-boost influenza vaccination are shaped by antigenic relatedness to priming strains. mBio 12 , e0044921 (2021).
Kim, J. H., Davis, W. G., Sambhara, S. & Jacob, J. Strategies to alleviate original antigenic sin responses to influenza viruses. Proc. Natl Acad. Sci. USA 109 , 13751–13756 (2012).
Download references
Acknowledgements
This work was supported by the National Natural Science Foundation of China (82304408); the Young Talents Project of Sichuan Academy of Medical Sciences and Sichuan Provincial People’s Hospital (2022QN08); the Youth Program of Science and Technology Department of Sichuan Province (24NSFSC2666); the Research Program of the Science and Technology Department of Sichuan Province (2022NSFSC0630); the Open Project of Central Nervous System Drug Key Laboratory of Sichuan Province (230015-01SZ) and the National Key Research and Development Program of China (2020YFC2005500).
Author information
These authors contributed equally: Yingying Hou, Min Chen, Yuan Bian.
Authors and Affiliations
Department of Pharmacy, Sichuan Academy of Medical Sciences & Sichuan Provincial People’s Hospital, School of Medicine, University of Electronic Science and Technology of China, Chengdu, 610072, China
Yingying Hou, Min Chen, Yuan Bian, Yuan Hu, Junlan Chuan, Lei Zhong, Yuxuan Zhu & Rongsheng Tong
Personalized Drug Therapy Key Laboratory of Sichuan Province, School of Medicine, University of Electronic Science and Technology of China, Chengdu, 610072, China
You can also search for this author in PubMed Google Scholar
Contributions
Y.Y.H. wrote the paper and drew the figures. M.C. and Y.B. wrote the paper. Y.H. and J.L.C. revised the paper. L.Z., Y.X.Z. and R.S.T. supervised the work. Y.Y.H. M.C. and Y.B. equally contributed to this study as co-first authors. All authors approved the final version of the manuscript.
Corresponding authors
Correspondence to Lei Zhong , Yuxuan Zhu or Rongsheng Tong .
Ethics declarations
Competing interests.
The authors declare no competing interests.
Additional information
Publisher’s note Springer Nature remains neutral with regard to jurisdictional claims in published maps and institutional affiliations.
Rights and permissions
Open Access This article is licensed under a Creative Commons Attribution 4.0 International License, which permits use, sharing, adaptation, distribution and reproduction in any medium or format, as long as you give appropriate credit to the original author(s) and the source, provide a link to the Creative Commons licence, and indicate if changes were made. The images or other third party material in this article are included in the article’s Creative Commons licence, unless indicated otherwise in a credit line to the material. If material is not included in the article’s Creative Commons licence and your intended use is not permitted by statutory regulation or exceeds the permitted use, you will need to obtain permission directly from the copyright holder. To view a copy of this licence, visit http://creativecommons.org/licenses/by/4.0/ .
Reprints and permissions
About this article
Cite this article.
Hou, Y., Chen, M., Bian, Y. et al. Insights into vaccines for elderly individuals: from the impacts of immunosenescence to delivery strategies. npj Vaccines 9 , 77 (2024). https://doi.org/10.1038/s41541-024-00874-4
Download citation
Received : 06 November 2023
Accepted : 28 March 2024
Published : 10 April 2024
DOI : https://doi.org/10.1038/s41541-024-00874-4
Share this article
Anyone you share the following link with will be able to read this content:
Sorry, a shareable link is not currently available for this article.
Provided by the Springer Nature SharedIt content-sharing initiative
Quick links
- Explore articles by subject
- Guide to authors
- Editorial policies
Sign up for the Nature Briefing: Translational Research newsletter — top stories in biotechnology, drug discovery and pharma.


An official website of the United States government
The .gov means it's official. Federal government websites often end in .gov or .mil. Before sharing sensitive information, make sure you're on a federal government site.
The site is secure. The https:// ensures that you are connecting to the official website and that any information you provide is encrypted and transmitted securely.
- Publications
- Account settings
- Browse Titles
NCBI Bookshelf. A service of the National Library of Medicine, National Institutes of Health.
Institute of Medicine (US) Forum on Emerging Infections; Knobler SL, Mahmoud AAF, Pray LA, editors. Biological Threats and Terrorism: Assessing The Science and Response Capabilities: Workshop Summary. Washington (DC): National Academies Press (US); 2002.

Biological Threats and Terrorism: Assessing The Science and Response Capabilities: Workshop Summary.
- Hardcopy Version at National Academies Press
3 Vaccines: Research, Development, Production, and Procurement Issues
Vaccines not only afford the best protection against infectious disease but can serve as strong deterrence factors as well. From a bioterrorist perspective, vaccine-resistant agents are more difficult to engineer than drug-resistant agents. But the potential market has been too small and uncertain to encourage the vaccine industry to make large investments in research, development, and manufacturing of new products. This is alarming considering the eight to ten years often needed to develop a new vaccine, compared to only two to three years to develop a new bioweapon.
Even among the four major vaccine manufacturers, there is insufficient production capacity. It was suggested during this session that in order to move animal and clinical testing forward, incentives need to be established to reduce the current challenges of vaccine development; vaccine production priorities need to be set and a central office or leader authorized to declare top priorities; and the role of the major vaccine manufacturers needs to be facilitated by clear directions and active collaboration between industry and government.
The use of vaccines as a civilian biodefense measure presents multiple challenges that are quite different from those of vaccine use by the military. Much of the challenge is due to the fact that the threats are uncertain and risk-benefit information difficult to assess. The very nature of terrorism produces a high level of uncertainty about what to expect and how to prepare. Additionally, DoD has developed vaccines to be used in normal healthy adults between the ages of 18 and 65, not pediatric, geriatric, immunocompromised or other subsets of the civilian population. Currently, there is no policy in place for immunizing the civilian population as a bioweapons defense measure, however several government agencies are working at unprecedented speed to put the correct policies into place.
The threat of a global pandemic makes smallpox one of the top vaccine priorities. An aggressive clinical development plan is currently in place; its goal is to build the stockpile with enough vaccine to protect the entire country within the year. The vaccine immune globulin (VIG) supply also needs to be expanded. Long-term goals include developing a safer vaccine that can be used in immunocompromised or other at-risk individuals.
Anthrax vaccine is another top priority. As of May 2001, over two million doses of the current anthrax vaccine have been administered to over 500,000 individuals, mostly military personnel. But there is an urgent need for more anthrax vaccine for the immunization of high risk civilian populations, as well as for use in medical management of exposed individuals in conjunction with antibiotics. Currently, there is only one manufacturer of licensed anthrax vaccine, but production is limited because of regulatory problems. Several commercial firms have offered to aid in scaled-up production, but the inherent variability of the manufacturing process and the risk of failure when scaling up so rapidly to such a high volume could create problems. Other mid to long-term anthrax vaccine needs include the development of a second-generation vaccine (e.g., a recombinant protective antigen vaccine) as well as better delivery technologies (e.g., plasmid DNA).
Of lesser importance than vaccines against smallpox and anthrax are vaccines against bacterial infections for which antibiotics can be used and other viral agents that, for the present, seem to be a lesser threat.
A recent independent review of DoD's vaccine acquisition program recommended an integrated approach between DoD and industry and the establishment of a dedicated national vaccine production facility that allows for maximal flexibility and expandable manufacturing capability for the production of various types of vaccines. Whether the proposed facility will be government-owned and contractor-operated or contractor-owned and contractor operated is open for discussion.
Ebola virus provides a useful paradigm for how a molecular-level understanding of the pathogenesis of a virus can be used to develop a new vaccine for an infectious agent that would otherwise be difficult to tackle. This type of molecular genetics approach can reveal possible targets for antiviral drugs as well. For example, recent studies have shown that one of the domains of the ebola virus forms a coil-to-coil structure that is similar to structures found in other viruses, including HIV and influenza. This similarity suggests that the approach being used to develop products for antiviral use against HIV may also be useful for targeting the coil-to-coil region of ebola virus. In fact, targeting this coil-to-coil structure may prove to be a useful general antiviral strategy against many different viruses.
Other vaccine issues that were raised during this session include:
- Improving the usefulness of DNA vaccines, which work well in rodents but not primates.
- Consideration of combination vaccines, for example can we use what we have learned from ebola to make a combination vaccine for use against all hemorrhagic fevers?
- Application of genomics to vaccine research could have, for example if we could use the new high throughput technology to identify genomic biomarkers for vaccine efficacy, then we could use these biomarkers in the future to move forward more quickly toward licensure.
- The need for a strong infrastructure to receive the intense flow of resources that would be expected with a rapid deployment of vaccines in response to out-breaks.
- The need for ways to accelerate vaccine FDA licensure without compromising product safety, for example use of the proposed animal efficacy rule for products that are either not feasible or ethical for human efficacy trials.
VACCINES FOR THREATENING AGENTS: ENSURING THE AVAILABILITY OF COUNTERMEASURES FOR BIOTERRORISM *
Affiliations.
Recent events have brought the subject of vaccines as a defense against bioterrorism into very sharp focus. We have been forced to take action in an area that, for the civilian sector, had previously been largely an academic debate and planning exercise with inadequate definitive action. We have changed from a nation of skeptics concerning the threat of bioterrorism to a nation of believers. Several government agencies are working at unprecedented speed to acquire the needed vaccines and put the correct policies into place for utilization.
However, the use of vaccines for defense against bioterrorism presents multiple challenges that are quite different from the traditional public health use of vaccines for protection against endemic or epidemic diseases. The issues are also quite different from those faced by the armed forces. The appropriate use of vaccines as a defense against bioterrorism presents major challenges in public policy development as well as public education. The ongoing public debates in the media highlight the complexity of the issues and reveal the widespread lack of understanding of the limitations of the current vaccines, especially vaccinia vaccine. For example, there is a call for widespread vaccination against smallpox but, in contrast, there is much misinformation and inappropriate fear about the effects of anthrax vaccine.
Some of the challenges involved with developing vaccine policies for defense against bioterrorism lie in the uncertainty of the threats. In contrast, policies for the use of vaccines against naturally-occurring disease threats are based on a wealth of historical and current epidemiologic information about disease burden and potential. Additionally, there is extensive data available on the safety of widely used vaccines that can be used to confidently assess risk benefit and cost effectiveness. In the case of agents of bioterrorism, however, risk assessment is much more difficult. The great difficulty in obtaining timely and reliable intelligence on the threat of biologic terrorism is a major part of the problem. Critical policy decisions—such as which vaccines will be needed, how large the stockpiles should be, and how the vaccine should be used—are greatly influenced by perceptions of threat. The very nature of terrorism produces a high level of uncertainty about what to expect and prepare for, and there is a wide and varying spectrum of perceived threats.
Obtaining the vaccines that are needed to protect our military and civilian populations depends entirely on effective government action. The potential market has been too small, at least up to the present time, to encourage the vaccine industry to make the large investments needed in research, development and manufacturing facilities. This has changed dramatically in the past two months. Nevertheless, the current situation is a result of past misjudgments, which resulted in insufficient government investment in vaccine research and development, and manufacturing capacity. There is an urgent need for rapid progress in R&D, manufacturing, and licensing processes, all of which are painfully slow processes when done by the usual methodologies.
Vaccines have varying usefulness in defense against bioterrorism. At the top of the list is the need for smallpox vaccine to prevent an outbreak from becoming a catastrophic global pandemic. Both smallpox and anthrax vaccines would be very useful in the medical management of exposed individuals, if the vaccines were readily available and placed in geographic proximity to multiple centers for distribution. Less important to the civilian populations are vaccines against bacterial agents that can be managed with antibiotics and viral agents which, at least for the present, seem to be lesser threats. These include plague, tularemia, hemorrhagic fever viruses, alphavirus encephalidities, Rift Valley fever, and others. However, several of these vaccines should be available for both civilian and military use. A government-owned production facility may be the best means for meeting the needs of these lower priority vaccines which will probably, at least initially, be made in much smaller quantities than smallpox and anthrax vaccines.
Smallpox Vaccine
The acquisition of a smallpox vaccine stockpile for civilian use started in 1999, with an Acambis contract for 40 million doses which now has been increased to 54 million doses. The seed virus was developed by cloning a New York City Board of Health strain derived from Wyeth Dry Vax. Animal model studies indicate that this strain appears to be somewhat less neurovirulent than the parent virus. The clinical development plan is aggressive; the phase I clinical trial should occur, as planned, in the latter part of January 2002. A very rapid procurement action has been in progress over the past weeks. The goal is to stockpile enough smallpox vaccine to protect the entire nation within the year. The response from the vaccine industry has been very heartening and has provided excellent options for utilizing existing manufacturing capacity to meet current requirements. Every effort will be made by CDC, FDA and NIH to assure that these contractors succeed to meet goals, time lines, and regulatory requirements. This will require truly unprecedented coordination and responsiveness by both the manufacturers and the various agencies.
Although the first step in building the smallpox vaccine stockpile is to ensure that vaccine manufacturing is underway, there are several other immediate issues that need to be addressed:
- Vaccination policy issues continue to be controversial. The CDC recently sent out a draft smallpox response plan to the states for comment. The plan calls for primary reliance on ring vaccination—the traditional method—to control an outbreak. The CDC has vaccinated 140 staff members who are most likely to be involved in investigating an outbreak, but no further vaccination with potential responders or health care providers is planned at this time. Laboratory personnel working with pox viruses will, of course, continue to be vaccinated.
- There is a need for more vaccine immune globulin (VIG) or VIG substitute to deal with the consequences of vaccination in immunosuppressed or other high risk subsets of the population. An interagency working group is currently exploring options for expanding the VIG supply.
- There is a need to develop a safer vaccine for use in immunosuppressed individuals, pregnant women, and other individuals for which the current vaccine is contraindicated. This will not only be a challenging research and development problem but also a challenging regulatory problem due to the difficulties in proving efficacy.
Anthrax Vaccine
The current licensed U.S. anthrax vaccine is a filtrate of culture media that contains a high level of PA (protective antigen) absorbed to alum; it probably contains small amounts of the other factors as well. An ongoing study at CDC is testing immunization schedules that involve fewer than the currently recommended six doses for this vaccine. Conventional wisdom has it that the live attenuated vaccines used in Russia and China are too reactogenic to be licensed in the United States. Israeli scientists have published reports on animal studies of experimental vaccines engineered to over-express recombinant protective antigen, but no clinical data are available.
The problems that the manufacturer has had with meeting regulatory criteria have limited the U.S. supply. A small amount of anthrax vaccine has been made available to DHHS by DoD, but that amount is far below what will be needed. There is an urgent need for a sufficient supply of anthrax vaccine for vaccinating high risk populations and for use as post-exposure vaccination in conjunction with antibiotics.
There are several immediate issues that need to be addressed. The production method for current licensed vaccine must be scaled up. Several commercial firms have made informal proposals to do this. However, this is a high risk option because of the inherent variability of the manufacturing process and the high risk of failure when scaling up so rapidly to such a high volume. There needs to be more serious consideration of the applications of the various platform technologies—such as plasmid DNA, viral vectors, and a variety of other delivery technologies—that are being developed within the biotech industry.
Finally, we need to accelerate development of a second generation vaccine. The time to availability could be shortened by overlapping large scale production with clinical trials. It has been suggested that we might have a stockpile of IND recombinant protective antigen (PA) vaccine within 18 months. This may be an achievable goal if all involved interests work in an effective, coordinated manner. A recombinant PA vaccine produced in E. coli will likely be the first to enter a phase I trial.
In order to address this issue of a second generation vaccine, the National Institute of Allergy and Infectious Diseases has put together a team with contractor help. Efforts are underway to gather all available information on ongoing or planned development efforts for a second generation anthrax vaccine, and compile the information in a systematic fashion and convene several advisors to review the resulting data, findings, and policy options. This may involve a major research and development contract program similar to what exists for smallpox vaccine and which will hopefully build on the work that has been done by DoD and DoD-DHHS collaboration. It will hopefully involve some new players as well, including the large vaccine manufacturers. Although it is difficult to predict which particular options will receive aggressive support, there is nonetheless a system now in place that will hopefully pave the way for pursuing an effective strategy in a reasonable period of time. The speed at which a second generation anthrax vaccine is developed will depend on both the underlying science and the responsiveness of the vaccine industry to national needs.
THE DEPARTMENT OF DEFENSE AND THE DEVELOPMENT AND PROCUREMENT OF VACCINES AGAINST DANGEROUS PATHOGENS: A ROLE IN THE MILITARY AND CIVILIAN SECTOR? *
Introduction.
In October 2001, the threat of bioterrorism became a reality. In support of this Forum's efforts to identify the obstacles to preparing an optimal response to bioterrorism—particularly as it relates to the complexities of interaction between private industry, research and public health agencies, regulatory agencies, policymakers, academic researchers, and the public—this paper will highlight emerging opportunities for more effective collaboration as well as scientific and programmatic needs for responding to bioterrorism. The focus of this paper is on the potential opportunities and issues related to Department of Defense (DoD) support for the research, development, and production of biological defense vaccines for the military and civilian populations to protect against bioterrorist threats. This paper will address the following topics:
- Current medical biological defense research and development efforts;
- Current biological defense vaccine capabilities;
- Proposed national biological defense vaccine production facility; and,
- Issues related to the use of biological defense vaccines.
In accordance with Congressional direction, DoD established a Joint Service Chemical and Biological Defense Program in 1994. The vision of the program is to ensure U.S. military personnel are the best equipped and best prepared force in the world for operating in future battlespaces that may feature chemical or biological contamination. The capabilities being developed for the military may have applicability to protection of civilians, especially as the military mission may increasingly support homeland security. Vaccines to protect against biological agents provide one critical capability to protect against the threat.
Medical Biological Defense Research and Development Efforts
The primary research program for the development of biological defense vaccines to protect U.S. forces is the Medical Biological Defense Research Program (MBDRP). In developing countermeasures to biological agents, the MBDRP uses a technical approach that focuses on four areas:
- Identify mechanisms involved in disease process;
- Develop and evaluate products (vaccines or drugs) to prevent or counter effects of toxins, bacteria, viruses, and genetically engineered threats;
- Develop methods to measure effectiveness of countermeasures in animal models that predict human response; and,
- Develop diagnostic systems and reagents
Biological defense vaccines are being developed to counter viruses, toxins, bacteria, and genetically engineered biological threat agents. Research activities start with basic research activities and proceed through the following steps, as research demonstrates successful candidates: (1) construction of the infectious clone, (2) identification of attenuating mutations, (3) construction of vaccine candidates, (4) testing in rodent models, (5) testing in non-human primates, (6) final selection, and (7) formulation. The formulated production may then become a candidate for an Investigational New Drug (IND) application for transition to advanced development and clinical trials, then ultimately licensed production.
An example of a product being developed within the MBDRP is the Next Generation Anthrax Vaccine. In cooperation with the National Institutes of Health, the next generation vaccine will provide greater or equal protection, require fewer doses to produce immunity, and have fewer adverse effects than the current anthrax vaccine. The reduced number of doses would provide greater flexibility to military forces by reducing the time constraint for developing immunity, hence accelerating the time for fielding a protected force. The next generation vaccine is based on recombinant protective antigen (rPA), which binds to the lethal factor (LF) and edema factor (EF) of B. anthracis . The recombinant production technology would eliminate need for spore-forming anthrax, and hence the need for a dedicated production facility. Overall, the next generation anthrax vaccine would decrease production cost, allow a greater range of potential vaccine production facilities, and potentially allow for streamlining of the regulatory approval process.
Another example of a product being developed within the MBDRP is Multiagent Vaccines (MAV) for Biological Warfare (BW) Threat Agents. The MAV project is a proof-of-principle effort to construct a vaccine or vaccine delivery approach that could concurrently immunize an individual against a range of BW threats. Bioengineered and recombinant vaccine technologies will be exploited to achieve vaccines that are directed against multiple agents, yet use the same basic construct for all of the agents. The MAV would be analogous to commercial vaccines (e.g., measles-mumps-rubella) but would exploit new approaches—naked DNA vaccines and replicon vaccines. The MAV would result in a reduced number of doses and thus provide greater flexibility to military forces by reducing the time constraint for developing immunity, hence accelerating the time for fielding a protected force. The MAV also could decrease production cost, allow for greater range of potential vaccine production facilities, and potentially allow for streamlining of the regulatory approval process.
Current Biological Defense Vaccine Capabilities
Joint vaccine acquisition program (jvap).
In order to enable the transition of candidate biological defense vaccines developed under the MBDRP or from other sources, a Prime Systems Contract was awarded in November 1997 to DynPort Vaccine Production Corporation, LLC. The JVAP was established for the purpose of developing, testing, and Food and Drug Administration (FDA) licensure of vaccine candidates, and production and storage of vaccine stockpiles. A major objective of the program is to establish a viable industrial base for vaccine production. The next generation anthrax vaccine (rPA) is one of several vaccines being investigated for development by the JVAP. Other vaccines in advanced development include smallpox, pentavalent Botulinum Toxoid, and tularemia. The Prime Systems Contract also provides options for other biological defense vaccines. Currently, all vaccines in the JVAP are in the development phase.
Anthrax Vaccine Adsorbed (AVA) and the Anthrax Vaccine Immunization Program (AVIP)
The only vaccine currently licensed for use in the United States to protect against anthrax is AVA. AVA is cell-free filtrate, produced by an avirulent strain of Bacillus anthracis . It is manufactured by BioPort Corporation in Lansing, Michigan and procured under a separate contract. It was licensed by the FDA in 1970. Six doses of the vaccine are required for full immunity, including doses at 0, 2, and 4 weeks, 6, 12, and 18 months, followed by an annual booster.
On December 15, 1997, the Secretary of Defense approved the decision to vaccinate all of the U.S. armed forces against anthrax, contingent on the successful completion of four conditions, which were met: supplemental testing of the vaccine; tracking of immunizations; approved operational and communications plans; and review of health and medical aspects of the program by an independent expert. Implementation is determined in accordance with DoD Directive 6205.3, “DoD Immunization Program for Biological Warfare Defense,” November 26, 1993, with complete implementation of the plan contingent upon adequate supply of the licensed vaccine
On May 28, 1998, the Secretary of Defense directed vaccination of the total force. Implementation of this directive was administered by the AVIP. As of May 29, 2001, more than two million doses were administered to more than 500,000 military personnel, with at least 70,000 completing the full six-shot regimen. Since then, there has been only a few who have received vaccinations. As outlined in a June 8, 2001 memorandum, the Secretary of the Army ordered a slowdown in immunization to accommodate delays in release of vaccine pending FDA approval. Implementation of the vaccination continues to designated special mission units, to vaccine manufacturing and DoD personnel conducting anthrax research, and others conducting Congressionally mandated anthrax vaccine research. Detailed information on the status of the AVIP is available at www.anthrax.osd.mil .
What Does Producing a Vaccine Mean?
With no vaccines currently in production under the JVAP and AVA as the only currently available FDA licensed vaccine for protection against BW threats, DoD is evaluating other mechanisms to increase and sustain vaccine production. In order to identify the status of vaccines, it is important to understand the major phases of research, development, and production through which they must proceed. Within different phases of vaccine development and production, there will be varying levels of production risk and overall risk. There are three major phases in the development and production of new vaccines—science and technology base, development and licensure, and licensed production. Following is a summary comparing different activities within each phase.
Production Approach
Within the science and technology phase, production is focused on small quantities and relies on bench top methods, which may include many different approaches, including new state-of-the-art experimental approaches. When a candidate product transitions to the next phase, a best approach is selected (or in some cases two or three promising approaches) and tested for scale up for full scale production. Following licensure, production proceeds at full scale and relies on a single, fixed method. Changes in the method typically require further testing and require approval by the FDA.
Vaccine Recipients
Perhaps the most obvious difference among the phases are the numbers and types of vaccine recipients and the purposes for which they receive the vaccine. Within the science and technology phase, recipients are primarily laboratory animals and include hundreds of animals. The primary purpose for using these recipients is to demonstrate the potential effectiveness of a vaccine candidate, that is proof-of-principle testing. During the development and licensure phase, vaccine recipients are humans, who participate in clinical trials. All recipients are volunteers, who participate in clinical trials that comply with FDA regulations. The focus of these investigations is to determine the safety and efficacy of a vaccine as well as to optimize dosing and scheduling. The final phase is production and includes providing a licensed vaccine to all individuals who may be at risk, in accordance with the FDA license and based on quantities available, for the purpose of providing protection against potential threats. The effected populations could be on the order of millions of individuals.
Production Risk
Production risk during the science and technology phase is moderate since only small quantities can be produced yet only small quantities are needed. Risk is minimized since FDA approval of the product is not required. During the development and licensure phase, production risk is usually high because of the risks involved in scaling up pilot lot product to full scale production. Overall risk is also high because of reliance on and surrogate models or biomarkers to determine efficacy, since law prohibits exposure of humans to chemical or biological agents.
Overall Risk
Overall risk for production of biological defense vaccines will vary depending on the type of vaccine being produced and the policy implemented for immunization. For example, use of a live vaccine (e.g., vaccinia live vaccine) poses risk that inoculated individual may be giving off live vaccinia viruses until scarification has occurred (2–5 days), hence potentially exposing unprotected individuals. Another risk is that low rates of adverse effects may become more apparent in a large scale immunization program than had occurred during testing. For example, if 1,000 people are tested in clinical trials and only one had a serious adverse reaction, there may be hundreds of reactions if the total military force is vaccinated.
Biological Defense Vaccine Development and Production Issues
One of the major factors limiting the availability of biological defense vaccines is the limited interest from the pharmaceutical industry in supporting the production of these vaccines. In contrast to vaccines to support public health needs (e.g., childhood diseases, influenza), most vaccine needs are fulfilled by the private sector. However, the private sector has some challenges in fulfilling public health vaccine needs. The vaccine production industrial base is nearly at full capacity to meet public health priorities. This will pose a challenge for the production of biological defense vaccines if production of biological defense vaccines results in the deferral of production of public health vaccines. Biological defense vaccines are considered specialty biologics and interest is primarily centered on a few small to mid-sized companies. Industry interest is limited in part because of requirements to conduct large, complicated clinical studies to demonstrate safety, immunogenicity, and efficacy (where possible).
Another major factor effecting the timely availability of biological defense vaccines are issues related to compliance with Chapter 21 of the Code of Federal Regulations (21 CFR), Food and Drug Administration (FDA). The specific issue relates to the ability to determine the clinical efficacy of biological defense vaccines. 21 CFR requires that for efficacy to be established, vaccines must be tested in informed, volunteer human subject who are exposed to the condition against which the vaccine is intended to protect. However, legal and ethical constraints prohibit exposing human subjects to biological agents. This constraint plus limited availability of human data for most vaccines mean that under current regulations, biological defense vaccine efficacy cannot be established. In order to address this constraint, FDA published a proposed rule on October 5, 1999 entitled, “New Drug and Biological Products; Evidence Needed to Demonstrate Efficacy of New Drugs for Use Against Lethal or Permanently Disabling Toxic Substances When Efficacy Studies in Humans Ethically Cannot Be Conducted; Proposed Rule.” (FDA rules are available at http://www.fda.gov/cber/rules.htm .) The proposed rule is expected to be finalized during 2002. Under this rule, efficacy may be determined based on data from clinical testing on animals (using at least two different species with preference that non-human primates be one of the species.) Animal data would serve as a surrogate for human data, but there would need to be significant data demonstrating that the effects in animals is related to effects in humans. Without the ability to license vaccines based on surrogate test data, biological defense vaccines would remain as investigational new drugs, which would continue to limit availability.
Proposed National Biological Defense Vaccine Production Facility
Following years of research, development, and efforts to produce biological defense vaccines in sufficient quantities to meet DoD needs, a different approach is currently being planned. In July 2001, DoD submitted a report to Congress detailing biological defense vaccine efforts within DoD. Known as the “Top Report”—because it provides the results of an independent expert panel chaired by Franklin Top, M.D.—this report summarized key shortcomings of current biological defense vaccine acquisition efforts. The report made the following findings and recommendations:
- The scope and complexity of the DoD biological warfare defense requirements are too great for either the DoD or the pharmaceutical industry to accomplish alone,
- The panel recommended a combined integrated approach whereupon DoD would work closely with the vaccine industry and national scientific base, and
- The panel recommended the construction of a government-owned, contractor operated (GOCO) vaccine production facility, which would include production capacity for up to eight vaccines over the next 7–12 years and would cost an estimated $2.4–$3.2 billion over that time.
The report recognized that in order for the GOCO to be successful, it would require long-term government commitment, increased resources, innovative DoD business and program management practices, and effective participation by established pharmaceutical industry leaders in vaccine discovery, licensure, and manufacturing.
The design concept for a GOCO biological defense vaccine production facility would accommodate three bulk vaccine production suites, each with different processes: spore-forming bacteria (for which FDA requires separate facilities), microbial fermentation, and tissue culture (viral vaccines). A modular design would allow flexible and expandable manufacturing capacity for production of DoD-cntical vaccines that are intended for force health protection.
The scale of the facility will be determined in part by the quantity of vaccines to be produced. The assumptions for the production capacity requirement are categorized into three tiers. Tier 1 is the baseline requirement and reflects current production requirements, which is the same as current requirements for the JVAP and AVIP. This tier includes sufficient anthrax vaccine for the entire force (approximately 2.4 million doses). It additionally would require 300,000 Troop Equivalent Doses (TEDs) for other biological defense vaccines. (Troop equivalent dose is defined as the number of vaccine administrations to reach full immunity. Boosters are not included.) Tier 2 would require three million TEDs (2.4 million for U.S. forces + 0.6 million for Commanders Reserve) of each vaccine to be produced to allow for total force protection plus sufficient quantities to support annual requirements due to personnel turnover. This requirement was the basis for the initial GOCO cost estimate. Tier 3 would require approximately 300 million TEDs of each vaccine to support civilian protection for the entire U.S. population.
In order to define the requirements for vaccine production and to ensure that it addresses national, and not just DoD needs, an interagency advisory group has been established. Interagency participation has been led by DoD and the Department of Health and Human Services, with participation from several organizations (including the Office of Homeland Security) to ensure a broad perspective. Federal participation is essential since biological defense vaccine needs are not being met by private industry. No individual department has the sufficient, full-spectrum capability and capacity to support vaccine needs. A national vaccine authority may be essential to ensure interagency needs are addressed not only in the planning phase but also in implementation. The details of the national vaccine authority are being developed, though it is not likely to be established as a new agency.
Issues Related to the Use Of Biological Defense Vaccines
Why vaccinate vaccine use risk management decisions.
BW agents pose high risk to military forces and operations, and at least ten countries are pursuing offensive BW programs. Vaccines are the lowest risk, most effective form of protection against BW threats. Vaccines are more effective and have fewer adverse effects than antibiotics or other treatments following exposure. While masks may provide highly effective protection, they may impede performance and must be worn to provide protection. Vaccines enable force protection by providing continuous, long-lasting protection. In addition, there are currently no real-time BW detection systems available. While there are systems that provide the ability to detection respirable aerosols in near real-time, the best available systems today take 15–45 minutes to identify a specific BW agent.
Vaccines are unusual among medical products in that they are given to healthy people to keep them healthy. Table 3-1 shows several of the vaccines commonly given to protect against infectious diseases and contrasts them with the limited number of biological defense vaccines currently available. Biological agents that may be used as weapons may be naturally occurring but have a very low incidence of natural occurrence (at least in the United States.)
Selected infectious diseases vaccines and biological defense vaccines.
The risk assessment for using biological defense vaccines is different from naturally occurring infectious diseases ( Grabenstein and Wilson 1999 ). Because to vaccinate is based on potential risk of disease outbreak rather than actual incidences. Consequently, a proper risk assessment for biological defense vaccines should not be a trade-off assessment between the actual adverse effects of a vaccine vs. the actual adverse effects of the disease, but the actual adverse effects of a vaccine vs. the potential adverse effects of the disease.
The policies on the use of biological defense vaccines will affect biological defense vaccine manufacturing. The two basic options for immunization are stockpiling vaccines in anticipation of a specific contingency or routine use immunization to ensure continued general readiness. If vaccines are stockpiled, manufacturing must address issues related to maintaining the stockpile as a result of the limited shelf life of some vaccines. Additionally, if vaccines are produced in bulk, once the required quantities are produced, manufacturers must ensure that the facilities remain capable of retaining an FDA facility license when production is not ongoing.
The assessment of potential and actual effects may effect product development. For example, as polio has been significantly reduced as a result of extensive vaccination, the Centers for Disease Control have recommended use of the inactivated polio vaccine (IPV) rather than the oral polio vaccine (OPV). While OPV has greater efficacy, it is also linked with rare occurrences of vaccine-associated paralysis. As cases of polio have been virtually eliminated in the United States, the risk of rare occurrences of adverse effects of the vaccine has exceeded the risk of the occurrences and effects of the disease.
If biological defense vaccines are produced and planned for use—especially among civilians populations—vaccine development criteria may place greater emphasis on vaccine safety than on vaccine effectiveness. Risk assessments may be complicated by the fact that the limited industrial base capacity for biological defense vaccine production will likely result in only one vaccine being available for military and civilian use.
There are other key differences between the military and civilian populations that make risk assessment difficult. One factor is that biological defense vaccines made for the military population are intended for use only in healthy adults. By contrast, the general population will also include significant subgroups for which vaccine safety, efficacy, or dosing information may not be fully understood, including pediatrics, geriatrics, pregnant women, and immune-compromised individuals. Currently there is no policy in place to immunize the civilian population absent a naturally occurring threat. If a licensed biological defense vaccine were available for use by the general population, an immunization policy for civilian use would be needed to address several issues before immunization could begin. Some of the issues that would need to be addressed are, for example, who would be vaccinated—the entire population, or a subgroup? Which subgroup(s)? Those living in specific regions? First responders? If symptoms of biological agent do not appear, would that be interpreted as the absence of a threat or the effectiveness of the defense? Paradoxically, would the demand for the vaccine diminish as the apparent threat also diminished? Civilians may also have greater concerns about the long term safety effects as a result of vaccine use. Additionally, there may be concerns regarding the unknown safety of the use of biological defense vaccines when interacting with other medical products. While there is no adequate basis to assess safety, there is no basis for extraordinary concern ( Institute of Medicine, 1996 ).
Conclusions
The Department of Defense may bring valuable assets to bear to counter the use of biological agents by terrorists. Currently, the DoD mission is focused on responding to threats to the military. Because of DoD's experience in defending against biological threats, DoD will continue to play a role in addressing the threat to the civilian population as well. DoD will continue to work with other agencies, including the new Director of Homeland Security, to determine what role it will play in homeland security, which will be defined in The Federal Response Plan, presidential directives, and other sources.
The availability of vaccine to protect against anthrax and other biological agents is based on several factors. One key factor is sustained resources to transition products from the science and technology base to advanced development. Resources include not only adequate funding, but also trained personnel, which is a critical factor since the biotechnology and pharmaceutical industry as a whole is facing shortages of skilled personnel. A second factor limiting the availability of biological defense vaccines is that they are similar to orphan drugs. There is no commercial incentive for manufacturers to produce vaccines. Federal investment may be required to retain the services and capabilities of the biotechnology and pharmaceutical industry.
While the availability of vaccines is critical, the decisions of whether to vaccinate will remain equally important. Vaccination decisions will continue to have greater physiological consequences than non-medical measures to protection against the threat (e.g., whether to wear masks). The decision will need to weigh the risk of actual low rates of adverse effects against the potential for protecting against catastrophic effects. In making these decisions based on risk, communicating the risk decision will be at least as important as risk assessment. Failure to have a coordinated public policy decision on vaccination support for civilians may result in individuals self-prescribing treatments or failing to comply with recommended guidelines.
APPLICATIONS OF MODERN TECHNOLOGY TO EMERGING INFECTIONS AND DISEASE DEVELOPMENT: A CASE STUDY OF EBOLA VIRUS *
In recent years, increasing attention has been focused on the Ebola virus as a potential public health problem, either from natural or deliberate outbreaks. Like the genetically related Marburg virus, Ebola is a filovirus that causes highly lethal hemorrhagic fever in humans and primates. Infection rapidly progresses from flu-like symptoms to hemorrhage, fever, hypotensive shock, and eventually, in about 50–90% of cases, death ( Peters et al., 1996 ; Peters and Khan, 1999 ). The molecular mechanisms underlying the pathogenicity of the Ebola virus are not well understood, in part because it has emerged only relatively recently (for reviews see Balter, 2000 ; Colebunders and Borchert, 2000 ). There was a series of outbreaks in central Africa in the mid-1970s and again in the 1990s (i.e., the Ivory Coast in 1994, Gabon in 1994–1996, Zaire in 1995, Gulu, Uganda in 2000 and presently in Gabon and the Republic of Congo). Ebola virus infection has appeared once in the United States, in Reston, Virginia. The Reston strain is not pathogenic in humans, and the outbreak was fortunately restricted to non-human primates.
One of the reasons that Ebola is highly lethal is that this virus replicates at an overwhelming rate ( Sanchez et al., 1996a ). Thousands of Ebola virus particles per host cell can completely envelop the cell and take over its entire protein synthetic machinery. We have only recently begun to understand the molecular mechanisms underlying this phenomenon. Although we have a descriptive understanding of the cytopathic effects of viral replication, we lack a clear understanding of how these various changes in cell structure and viability occur. Elucidating these details will be critical for developing vaccines and other antiviral therapies.
Aside from the obvious immediate health threat that would be posed if it were introduced into the population, Ebola virus represents a useful paradigm for dissecting the molecular genetics of a virus. Most of what is known about Ebola pathogenesis is derived from genetic studies of the virus. Although Ebola is very similar to the genetically related Marburg virus, it differs in at least one important respect. The gene that encodes the viral glycoprotein in Ebola generates two gene products, whereas in Marburg, this gene encodes a single protein (Sanchez et al., 1996). One of the gene products is secreted as a soluble 50 to 70 kDa glycoprotein, whereas the other is a full-length 120 to 150 kDa glycoprotein that inserts into the viral membrane ( Volchkov et al., 1995 ; Sanchez et al., 1996). The secreted form was originally believed to serve as an immunological decoy for the full-length glycoprotein, allowing the full-length glycoprotein to attach to the target cell. However, more recent evidence now suggests that this hypothesis is unlikely. Instead, the secreted form appears to inhibit early steps in neutrophil activation and thereby inhibit the host inflammatory response to the virus ( Yang et al., 1998 ). The secreted glycoproteins have been shown to bind quite well to neutrophils, but bind poorly to endothelial cells ( Yang et al., 1998 ). In contrast, the full-length glycoprotein interacts with endothelial cells but binds poorly to neutrophils ( Yang et al., 1998 ). This glycoprotein enables the Ebola virus to recognize and introduce its viral contents into the endothelial cell lining of the blood vessels, as well as monocytes/macrophages, thereby resulting in the cellular damage that is associated with the devastating symptoms of Ebola infection.
Antiviral Targets
Detailed analyses of the mechanisms of viral entry, replication, and cell damage have identified the Ebola glycoprotein 2 (GP2) as a potential antiviral target. In particular, there is a region in the GP2 ectodomain of Ebola virus that forms a coiled coil, or hairpin-like structure similar to what exists in the human immunodeficiency virus (HIV), influenza, respiratory syncytial virus, and a variety of other viruses ( Weissenhorn et al., 1998a , 1998b ; Malashkevich et al., 1999 ). This coiled-coil region contributes to membrane fusion by undergoing conformational changes after the glycoprotein binds to the membrane receptor ( Weissenhorn et al., 1998b ; Watanabe et al., 2000 ). The fact that this structure is conserved in a number of different viruses suggests that it may represent a potential target for antiviral therapy. In fact, a peptide product directed at the analogous structure in HIV has potent antiviral effects and is currently being developed for the clinical treatment of AIDS. This or similar peptides could be useful against many other viruses as well, including Ebola.
Not only does the transmembrane glycoprotein direct the Ebola virus into specific cells, but the glycoprotein itself is also highly toxic to cells. For example, when full-length Ebola glycoprotein is overexpressed in cultured renal epithelial cells, it inserts into the membrane and causes morphological changes and detachment from culture dishes ( Yang et al., 2000 ). This finding suggests that there is a genetic determinant in the glycoprotein that mediates its toxicity and, therefore, might represent another potential target for antiviral therapies. Mapping studies identified a serine-threonine-rich, mucin-like core domain region of the glycoprotein that is required for cytotoxicity in human endothelial cells ( Yang et al., 2000 ). When the mucin-like region of the glycoprotein was deleted, its cytotoxicity was abolished, but protein expression and function remained unchanged ( Yang et al., 2000 ). Every possible open reading frame in the Ebola virus genome has been tested for toxicity, except for the polymerase region. To date, only the glycoprotein has been shown to induce toxic cytopathic changes. However, a better understanding of the detailed molecular mechanism of virus assembly may eventually provide insight into other potential antiviral targets as well.
Vaccine Development
Not only does the glycoprotein play an important role in toxicity, increasing evidence suggests that it also plays an important role in the pathogenesis of Ebola infection. Infection of cultured cells with adenoviral vectors encoding the glycoprotein causes considerable cellular damage that correlates with toxicity. However, overexpression of a glycoprotein that is unable to insert into the cell membrane is not cytotoxic. In fact, injecting adenoviral vectors, or DNA forms of these vectors, into mice, rabbits, and primates actually protects the animals from disease by inducing an effective vaccine response. No human vaccine against Ebola is currently available. However, studies in animals suggest that DNA vaccines, together with replication-defective adenoviral vectors, may be particularly promising. In the DNA vaccination platform, a plasmid expression vector is injected into muscle, thereby enabling muscle to synthesize large quantities of proteins that stimulate the immune system to generate an effective immune response. DNA vaccination technology could greatly simplify the vaccination production process that would otherwise rely on very large-scale plants for making these complex and highly purified proteins. However, although current DNA vaccines work well in rodents, they are not as effective in non-human primates and are even less robust in humans. Thus, one of the important challenges for developing an effective DNA vaccination platform technology is to improve immune responses in non-human primates and humans.
The first successful studies of a DNA vaccine for Ebola virus were carried out in guinea pigs (Xu et al., 1999). Animals that were immunized with sufficient levels of Ebola virus glycoprotein to induce a high-titer antibody response survived infection. Guinea pigs with intermediate levels of titers exhibited an intermediate chance of survival. In contrast, none of the control animals, immunized with vector alone, survived Ebola infection (Xu et al., 1999). “Prime-boost” strategies combine DNA immunization and boosting with adenoviral vectors that encode viral proteins to specifically target dendritic cells. Such DNA vector-viral vector combinations can be very potent. Animals are first immunized with a DNA vector, and typically develop titers ranging from 1:1,500 to about 1:3,500. Following the adenovirus boost, antibody titers increase dramatically, ranging from 1:50,000 to 1:100,000. This far exceeds the minimum threshold that is considered to be necessary for an effective immune response in primates. A modified prime-boost strategy was recently used to immunize cynomolgus macaques against several strains of the Ebola glycoprotein ( Sullivan et al., 2000 ). Several months later, animals were boosted with recombinant adenovirus expressing the Ebola (Zaire) glycoprotein. Control animals received empty vectors consisting of plasmid DNA and ADV-DE1 recombinant adenovirus in a parallel injection regimen. When animals were subsequently challenged with a lethal dose of the Zaire subtype of Ebola virus, all control animals (6 out of 6) exhibited rapid increases in their viral antigen levels and succumbed to infection within seven days. In striking contrast, all animals immunized with the combination DNA-adenovirus vaccine survived Ebola virus challenge (4 out of 4). The level of antibody production and the cellular proliferative response were closely correlated with immunoprotection.
It is of interest to note that vaccines are not only clinically useful, but they can also serve an important function as deterrents against bioweapons. It is much more difficult to engineer vaccine resistance than drug resistance in an organism. Having well-defined, publicly known, and effective vaccines is a critical preventive, or deterrent, strategy. Another benefit of a successful vaccine is that it opens the way for developing novel immunotherapies. In the case of Ebola virus, for example, hyperimmune serum from animals that are protected from the disease is currently being examined, to determine if it can be used during the course of infection as a possible post-exposure therapy.
Role of Genomics in Vaccine Development and Biodefense
Genomic approaches hold enormous potential for vaccine development, and these possibilities are only just beginning to be explored. For example:
- Analysis of global gene expression patterns can facilitate the early identification of both environmental and disease-associated pathogens.
- Gene expression patterns can be used to identify specific genetic susceptibility and resistance markers.
- Biomarkers for vaccine efficacy could be incorporated into the experimental design of efficacy trials, which could then accelerate approval and licensure processes.
- High throughput technology can be used to improve vaccine design, by allowing researchers to readily monitor how specific structural changes in the vaccine affect the cellular response to immunization.
It is possible that enough information will eventually be available and implemented within the technology that simply knowing the sequence of a particular open reading frame will be sufficient to understand how to generate an effective vaccine. Such technology would be useful not only as a defense measure against bioterroism, but also for the prevention or treatment of naturally occurring outbreaks, such as influenza. The influenza virus constantly mutates, but if genetic information could be acquired quickly enough, it may become possible to develop more effective countermeasures.
In conclusion, the process of vaccine development must evolve to become more responsive to the changing needs and emerging outbreaks of society today. In other words, more agile vaccines are needed. Agility includes the ability to rapidly deploy vaccines in the event of an outbreak; to accelerate immunization regimens so that such an outbreak could be effectively managed; and, finally, new technology must be applied to develop better vaccines and to accelerate the development process.
MEETING THE REGULATORY AND PRODUCT DEVELOPMENT CHALLENGES FOR VACCINES AND OTHER BIOLOGICS TO ADDRESS TERRORISM *
The FDA plays an important role in multiple stages of the product development process, from initial clinical studies through licensure, manufacturing and post-marketing studies which may be used to further evaluate safety and effectiveness. For these reasons, FDA is committed to working together with the scientific and clinical communities and with industry and the public to fulfill its regulatory and public health role in facilitating the development of biodefense biologics and therapeutics. Recent and ongoing FDA biodefense-related activities include, for example, meeting with sponsors and sister agencies and departments to encourage interest in developing safe and effective new products needed for public health biodefense, performing research that ultimately facilitates the development of these products; and providing intensive and early interactions with product sponsors to speed their availability.
As with any medical product, bioterrorism products need to be regulated to ensure consistent and objective protection of the public safety. While there is currently a sense of emergency and a set of urgent needs to address, the desire for rapid and innovative responses must not be allowed to compromise the objective assessment of safety and effectiveness. Thus we need a regulatory agency that can step back and provide a more objective perspective. If and when things go wrong in the wake of decision(s) made in a time of crisis, few people will remember the crisis and that the decision was in fact made with the best intentions. The public expects safe and effective products, and safety expectations are especially high for vaccines administered to healthy individuals. Maintaining public confidence in vaccines and medical products, in general, is critical to maintaining overall confidence in our nation's public health programs and leadership in matters extending far beyond bioterrorism. For these reasons, even in difficult times, we must continue to make and communicate clearly the best possible scientific and public health decisions about product development, licensure, availability and use.
Furthermore, bioterrorism is a moving target, not a single disease of predictable epidemiology, and all potential product uses may not be anticipated. This complicates many decisions about product use. For example, a vaccine, such as the licensed anthrax vaccine, which may have been originally studied and used in a limited population effectively and without major safety concerns may raise more significant public concerns about uncommon adverse events, whether coincidental or due to the vaccine, if and when it is administered for similar reasons to hundreds of thousands of people or when unanticipatedly used for post-exposure prophylaxis.
There are several factors that account for why we do not have an adequate supply of vaccines for bioterrorism defense:
Financial Disincentives
- Uncertain markets, especially for potentially more limited use products such as a tularemia or plague vaccine.
- Uncertain longevity of the needs, markets and of resources; short attention spans in government budgeting.
- The fact that vaccines are complex biological products that carry a high risk of uncertainty, unpredictability of success, and financial loss.
- The rigorous safety requirements and low public tolerance of risk—in part because they are often administered to healthy people as a preventative measure—and associated costs of developing biologics.
- The fact that preventive measures are generally undervalued, both perceptually and financially. Vaccines are often expected to be sold for very low prices, and the expected profit for the producer is therefore lower than for other products (e.g., drugs for treatment) competing for the same resources. However, while difficult to model when risks are unclear, it would be interesting to conduct more comprehensive and long-term cost-benefit analyses concerning the personal health impacts and the social and economic costs versus potential benefits of vaccine compared to treatment strategies for specific agents of interest.
- The added cost of the large clinical trials needed to address potential wide use including in diverse populations.
- The presence of advocacy groups with various points of view.
- A fair amount of concern about possible adverse effects of vaccines, ranging from specific disease issues to more general anti-vaccine sentiment on the part of a proportion of the public.
- A mistrust of government and industry
- Product liability issues.
Scientific Challenges
- Lack of historical or recent precedents for vaccines against many pathogens, which makes it difficult to establish good surrogates.
- The potential for genetic or other manipulation of antigenic determinants. (Although this is presently more difficult in many cases to engineer than antibacterial resistance.)
- The potential complications of live vaccine administration to increasing immunocompromised populations.
- The intense flow of resources demanded by urgent perceived needs (sometimes referred to as the “disease du jour” phenomenon), in contrast to the more normal lengthy product development cycle.
The FDA Response
There are several regulatory approaches and mechanisms that the FDA has employed in an attempt to safely speed up product availability and licensure:
- Early and frequent consultation between the sponsor producing the product, the potential end users (e.g., health officials and providers in the military and civilian sectors), and the FDA is very resource-intensive but important. This kind of up-front investment can greatly improve the product development process by identifying creative study designs, recognizing factors that are normally not anticipated in developing a product, and reducing misunderstandings and the likelihood of unwelcome surprises. Early dialogue also increases accountability.
- Emergency use under IND (investigational new drug status) allows rapid access to products that have not yet completed requirements for licensure. INDs require acceptable evidence of safety; a reasonable though not necessarily formally proven scientific basis for efficacy; a favorable nsk:benefit ratio; and an intent to license. While allowing availability of potentially lifesavmg products, a disadvantage to emergency use under this rule is that the product is not licensed, which not only reflects the true scientific limitations of the data but also raises important issues about public perception.
- Fast track processes can speed up the review process for products that will provide meaningful therapeutic benefits compared to existing therapies for serious or life-threatening illnesses. Fast track allows the FDA to review information as it becomes available and as the sponsor submits it.
- Accelerated approval through the use of surrogate end points to demonstrate benefit. The use of CD4 cells for assessment of antiviral treatment of HIV was one of the first surrogates to be approved under this rule. For bioterrorist agents, protective antibody levels for a vaccine or immunoglobulin could serve as potential surrogate end points. Clinical end points can also be utilized. There still must be good post-licensure studies to demonstrate the effects on disease outcomes and to collect additional safety information, and the FDA can place restrictions on use and promotion and even withdraw the product if agreements are violated or the product proves unsafe or ineffective. Thus far, this process has worked fairly well although, once a product is licensed, or if a disease is rare, it may be difficult to obtain patients for studies, and sponsors sometimes are unable or unmotivated to fulfill their commitments. But because most accelerated approval products also receive priority review, this process can allow for rapid approval of a product based on more limited and simpler-to-obtain clinical data than may be the case with large, randomized control trials and/or longer-term endpoints.
- Priority review is applied when a product is considered a significant advance or will be used for serious or life-threatening illness.
- Approval under the forthcoming “Animal Rule” has very important biodefense implications. In fact, the rule is specifically oriented to drugs or biologics that reduce or prevent serious or life threatening conditions caused by exposure to lethal or disabling toxic, chemical, biologic, or nuclear threats. The products should be expected to provide a meaningful therapeutic benefit over existing treatments. Human efficacy trials should either be not feasible or unethical, and the use of the animal efficacy data should be scientifically appropriate. In this proposed rule, the end point should be related to the desired benefit in humans, usually a significant outcome such as mortality or major morbidity. Clinical studies in representative populations are still needed, however, both for establishing pharmacokinetics (including, in the case of many vaccines, immunogenicity) and for assessing safety. Such studies are critical because civilian populations often include vulnerable or pharmacokinetically variable subsets. Finally, similar to the fast track and accelerated approvals, the animal rule has post-marketing and labeling commitments and restrictions. It does not apply if the product could be approved based on any other standard in FDA's regulation. It is a rule of last resort, but it certainly would be applicable to many of the situations that have been described in this workshop.
In addition to its regulatory responsibilities, the FDA's Center for Biologics conducts a significant amount of biodefense-related research, supporting approximately sixty ongoing projects that are directly relevant to identified high threat agents. The general goal is to meet otherwise unmet research needs, often with regulatory implications. Examples include how to better determine potency; defining immune and other correlates of protection; how to make safer and purer products (including characterization of the safety of cell substrates and detection of adventitious agents); better assessment of adverse events and efficacy under conditions of use, and studies which allow the agency to make regulations more scientific and less “defensive.” These types of research can benefit not only the public, but also multiple companies across industry, but are often not performed by a given sponsor as they may not provide a direct and/or immediate benefit. Furthermore, through its research and related scientific interactions, the center maintains the type of cutting edge expertise that is increasingly needed for dealing intelligently and proactively with evolving products and their underlying biotechnology. This expertise and confidence fosters the science-based objectivity necessary for anticipating and/or reacting appropriately to the issues raised during the development of a product which, ultimately, accelerates the regulatory and licensure process.
By maintaining its scientific, objective regulatory stance, the FDA can increase confidence in the likely efficacy of products primarily approved based on surrogate/animal data and reduce the likelihood of serious adverse events. The FDA brings several other unique attributes to the product development process as well, including:
- Knowledge of scientific and industrial capabilities, which is very helpful when it is necessary to identify people with specific expertise. This includes knowledge of emerging technologies which are cross-cutting among diverse products that nobody else may have the opportunity to see; knowledge of manufacturing capabilities; and knowledge of potential new uses of both licensed and investigational products, for example anti-sepsis and immune modulators.
- Day-to-day participation in what it takes to develop a product, including clinical trials, quality assurance, adverse event monitoring, timelines, etc.
- A unique ability to match product needs to industrial and academic capabilities. Much of this is informal, but it can be very helpful in getting the job done well.
However, there are several things that the FDA cannot do. FDA cannot
- provide monetary or tax incentives;
- assure that anyone will make a product;
- sponsor or directly assume the burden of product development, since this would be a conflict of interest;
- provide indemnification or compensation for injuries;
Furthermore, while the prelicensure process can provide reasonable assurances about the degree of safety and effectiveness, FDA cannot
- guarantee absolute safety;
- guarantee human efficacy under field conditions based on non-human data such as animal studies or surrogate endpoints (or, for that matter, based on efficacy observed in the controlled setting of a clinical trial).
In addition to expedited regulatory pathways, as well as orphan drug status, there are several potential incentives—both push and pull—which are outside the mission of FDA but that could be evaluated with respect to their potential to stimulate product development. Push incentives, which could be considered where markets are small or uncertain, could include:
- direct financial awards or contracts;
- tax credits;
- enhanced exclusivity;
- partnerships in product development; and
- research and development assistance to reduce the financial sting and risk of product development.
Possible pull incentives, which are probably more valuable, include:
- known markets;
- longer term financial contracts;
- defining prices that more accurately reflect known and potential public health benefit, which will require more economic discussion and modeling; and
- where possible, developing dual or multiple use products/concepts which can used not just for meeting bioterrorism needs but also for enhancing general public health and medical care.
In summary, FDA and CBER are highly committed to working with multiple partners in and outside of government to help in meeting the challenges posed by bioterrorism. Especially in times of threat and crisis, there is a need for a responsive, yet independent and science-based regulatory process. Relevant research and expertise remains critical in meeting the challenge. Existing laws and regulations can help facilitate product development in a timely manner. There are significant financial disincentives which have and may continue to impede the industrial development of some needed products where markets may be small or uncertain. Careful and open communication with the public about what is and is not known about proposed bioterrorism responses using new and existing products is critical not only in responding to specific threats and protecting the public but also in maintaining confidence in and support for the public health system as a whole.
- MOVING THE VACCINE AGENDA FORWARD: OBSTACLES AND OPPORTUNITIES
The vaccine industry is highly concentrated with only four major manufacturers providing more than three quarters of the market. Even within these four mam manufacturers production capacity may be insufficient for unseen circumstances or urgent need, as has been demonstrated by shortages of DT Acellular pertussis vaccine and pneumococcal conjugate vaccine. Following September 11th, all four major manufacturers expressed interest in biodefense/military vaccines. But how long will patriotism sustain this interest? The industry is both high risk (e.g., the rotavirus vaccine which had to be withdrawn from use) and risk adverse (e.g., certain vaccines for pregnant women have yet to be developed). There are several factors that impede vaccine product development:
- The vaccine industry is market-driven, and the major manufacturers are simply not interested if the market is insufficient.
- The industry is highly regulated, and perceived regulatory hurdles can impede product development.
- Intellectual property conflicts can prevent companies from developing products.
- Negative marketing assessment impedes vaccine production. Marketing departments often make unchecked predictions.
- An uncertain or dubious proof of concept creates reluctance to develop a particular new product.
- Biohazard to personnel, especially with regards to biodefense vaccines, can create reluctance.
The production of an adequate biodefense vaccine supply will depend on many factors:
- Development of biodefense vaccines must be in collaboration with DoD, NIH, CDC, and other agencies with national interests. With regard to the development of a new smallpox vaccine, for example, efficacy is an important issue that requires the use of monkey models for which the vaccine industry must turn to DoD or NIH.
- Development of biodefense vaccines requires better adverse reaction surveillance. With the military anthrax vaccine, for example, initially there were no data to support the claim that the vaccine was indeed safe. New vaccines must have built-in surveillance in order to discredit unsubstantiated claims about adverse reactions based on anecdotal data.
- New vaccine production requires that liability indemnification be guaranteed. One possible solution would be to place these vaccines on a list of compensable vaccines. We need to create a no-fault system which indemnifies companies against non-negligent harm but also provides some relief for injured individuals.
- Access to new technology would likely stimulate more interest in vaccine development. For example, a number of companies have pursued DNA vaccines because they are an interesting new technology which could likely be applied to a number of different targets. After all, the mam role of the vaccine industry is not to conduct basic research but apply basic research to the development of new products.
- Finally, vaccine production priorities need to be set and somebody authorized to say “this is the top priority.” For example, Gary Nabel has presented some very elegant work on an Ebola vaccine. But what does intelligence say is the real risk of Ebola? Should a virus that cannot be spread as an aerosol be considered a priority?
In summary, the major vaccine manufacturers will not be able to provide all of the needed biodefense vaccines. However, they should be asked to play a significant role. That role will be facilitated by clear directions, clear priority setting, and a tight collaboration between industry and government in order to move animal and clinical testing forward.
A. Johnson-Winegar
- Grabenstein JD, Wilson JP. Are Vaccines Safe? Risk Communication Applied to Vaccination. Hospital Pharmacy. 1999; 34 (6):713–729.
- Institute of Medicine. Interaction of Drugs, Biologics, and Chemicals in U.S. Military Forces. Washington, D.C.: National Academy Press; 1996. [ PubMed : 25121292 ]
- Use of Anthrax Vaccine in the United States—Recommendations of the Advisory Committee on Immunization Practices. Morbidity and Mortality Weekly Report. 2000 December 15; 49 (RR15):1–20. [ PubMed : 11145529 ]
- Balter M. Science. 2000; 290 :923–925. [ PubMed : 11184732 ]
- Colebunders R, Borchert M. Journal of Infections. 2000; 40 :16–20. [ PubMed : 10762106 ]
- Malashkevich VN, Schneider BJ, McNally ML, Milhollen MA, Pang JX, Kim PS. Proceeding of the National Academy of Sciences, U S A. 1999; 96 :2662–2667. [ PMC free article : PMC15825 ] [ PubMed : 10077567 ]
- Peters CJ, Sanchez A, Rollin PE, Ksiazek TG, Murphy FA. Fields Virology. Fields BN, Knipe DM, Howley PM, editors. Lippincott-Raven; Philadelphia: 1996. pp. 1161–1176.
- Peters CJ, Khan AS. Current Topics in Microbiology and Immunology. Klenk HD, editor. Vol. 235. Springer-Verlag; Berlin: 1999. pp. 85–95. [ PubMed : 9893380 ]
- Sanchez A, Khan AS, Zaki SR, Nabel GJ, Ksiazek TG, Peters CJ. Fields Virology. Fields BN, Knipe DM, Howley PM, editors. Lippincott-Raven; Philadelphia: 1996. pp. 1279–1304.
- Sanchez A, Trappier SG, Mahy BWJ, Peters CJ, Nichol ST. Proceedings of the National Academy of Sciences, USA. 1996; 93 :3602–3607. [ PMC free article : PMC39657 ] [ PubMed : 8622982 ]
- Sullivan NJ, Sanchez A, Rollin PE, Yang ZY, Nabel GJ. Nature. 2000; 408 :605–609. [ PubMed : 11117750 ]
- Volchkov VE, Becker S, Volchkova VA, Ternovoj VA, Kotov AN, Netesov SV, Klenk HD. Virology. 1995; 214 :421–430. [ PubMed : 8553543 ]
- Watanabe S, Takada A, Watanabe T, Ito H, Kida H, Kawaoka Y. Journal of Virology. 2000; 74 :10194–10201. [ PMC free article : PMC102058 ] [ PubMed : 11024148 ]
- Weissenhorn W, Calder LJ, Wharton SA, Skehel JJ, Wiley DC. Proceedings of the National Academy of Sciences, USA. 1998; 95 :6032–6036. [ PMC free article : PMC27580 ] [ PubMed : 9600912 ]
- Weissenhorn W, Carfi A, Lee KH, Skehel JJ, Wiley DC. Molecular Cell. 1998; 5 :605–616. [ PubMed : 9844633 ]
- Xu L, Sanchez A, Yang Z, Zaki SR, Nabel EG, Nichol ST, Nabel GJ. Nature Medicine. 1998; 4 :37–42. [ PubMed : 9427604 ]
- Yang Z, Delgado R, Xu L, Todd RF, Nabel EG, Sanchez A, Nabel GJ. Science. 1998; 279 :1034–1037. [ PubMed : 9461435 ]
- Yang ZY, Duckers HJ, Sullivan NJ, Sanchez A, Nabel EG, Nabel GJ. Nature Medicine. 2000; 6 :886–889. [ PubMed : 10932225 ]
This statement reflects the professional view of the author and should not be construed as an official position of the Department of Health and Human Services.
The information provided in this paper reflects the professional view of the author and not an official position of the U.S. Department of Defense.
This statement reflects the professional view of the author and should not be construed as an official position of the National Institute for Allergy and Infectious Diseases, National Institutes of Health.
This statement reflects the professional view of the author and should not be construed as an official position of the Food and Drug Administration.
- Cite this Page Institute of Medicine (US) Forum on Emerging Infections; Knobler SL, Mahmoud AAF, Pray LA, editors. Biological Threats and Terrorism: Assessing The Science and Response Capabilities: Workshop Summary. Washington (DC): National Academies Press (US); 2002. 3, Vaccines: Research, Development, Production, and Procurement Issues.
- PDF version of this title (5.5M)
In this Page
- VACCINES FOR THREATENING AGENTS: ENSURING THE AVAILABILITY OF COUNTERMEASURES FOR BIOTERRORISM
- THE DEPARTMENT OF DEFENSE AND THE DEVELOPMENT AND PROCUREMENT OF VACCINES AGAINST DANGEROUS PATHOGENS: A ROLE IN THE MILITARY AND CIVILIAN SECTOR?
- APPLICATIONS OF MODERN TECHNOLOGY TO EMERGING INFECTIONS AND DISEASE DEVELOPMENT: A CASE STUDY OF EBOLA VIRUS
- MEETING THE REGULATORY AND PRODUCT DEVELOPMENT CHALLENGES FOR VACCINES AND OTHER BIOLOGICS TO ADDRESS TERRORISM
Other titles in this collection
- The National Academies Collection: Reports funded by National Institutes of Health
Related information
- PMC PubMed Central citations
- PubMed Links to PubMed
Recent Activity
- Vaccines: Research, Development, Production, and Procurement Issues - Biological... Vaccines: Research, Development, Production, and Procurement Issues - Biological Threats and Terrorism
Your browsing activity is empty.
Activity recording is turned off.
Turn recording back on
Connect with NLM
National Library of Medicine 8600 Rockville Pike Bethesda, MD 20894
Web Policies FOIA HHS Vulnerability Disclosure
Help Accessibility Careers

IMAGES
VIDEO
COMMENTS
Tetanus vaccines: WHO position paper, February 2017 — recommendations. Vaccine 36, 3573-3575 ... (SAGE) and a National Institute for Health Research (NIHR) Senior Investigator. The views ...
A two-dose regimen of BNT162b2 (30 μg per dose, given 21 days apart) was found to be safe and 95% effective against Covid-19. The vaccine met both primary efficacy end points, with more than a 99 ...
Since the outbreak of the COVID-19 pandemic, there has been a rapid expansion in vaccine research focusing on exploiting the novel discoveries on the pathophysiology, genomics, and molecular biology of the severe acute respiratory syndrome coronavirus 2 (SARS-CoV-2) infection. Although the current preventive measures are primarily socially ...
Overall, the PHE study showed vaccine effectiveness after two doses of the BNT162b2 vaccine of 94% against the alpha variant and 88% against the delta variant. The corresponding percentages with ...
The protective effects of vaccination and prior infection against severe Covid-19 are reviewed, with proposed directions for future research, including mucosal immunity and intermittent vaccine boo...
Our analyses indicate that vaccine effectiveness generally decreases over time against SARS-CoV-2 infections, hospitalisations, and mortality. The baseline vaccine effectiveness levels for the omicron variant were notably lower than for other variants. Therefore, other preventive measures (eg, face-mask wearing and physical distancing) might be necessary to manage the pandemic in the long term.
The effectiveness of the mRNA vaccines in preventing COVID-19 disease progression in 2021 set new expectations about the role of prevention interventions for the disease. Efficacy observed in the trials was more than 90%.1,2 The efficacy of other vaccines evaluated in large randomised trials, such as the Oxford-AstraZeneca (70%) and Sputnik V (91%) vaccines, have been criticised for elements ...
2. Vaccination strategies. Many efforts have been directed towards the development of the vaccines against COVID-19, to avert the pandemic and most of the developing vaccine candidates have been using the S-protein of SARS-CoV-2 (Dhama et al., 2020).As of July 2, 2020, the worldwide SARS-CoV-2 vaccine landscape includes 158 vaccine candidates, out of which 135 are in the preclinical or the ...
To date, coronavirus disease 2019 (COVID-19) becomes increasingly fierce due to the emergence of variants. Rapid herd immunity through vaccination is needed to block the mutation and prevent the emergence of variants that can completely escape the immune surveillance. We aimed to systematically evaluate the effectiveness and safety of COVID-19 vaccines in the real world and to establish a ...
Despite questions remain about the impact of virus variants and the duration of the immune response, messenger RNA (mRNA)-based and adenoviral vectored vaccines have demonstrated an overall efficacy from 70 to 95% in both phase III trials and real life. In addition, all these vaccines also reduce the severe forms of the disease and might ...
Vaccination is a powerful method of disease prevention that is relevant to people of all ages and in all countries, as the Covid-19 pandemic illustrates. Vaccination can improve people's chances ...
A case-control research was carried out in Thailand by Sritipsukho et al. to assess the efficacy of various vaccination regimens in preventing COVID-19 during the time when the delta variant was the predominant causing virus (≥ 95%). By correcting for individual demographic and clinical factors, the efficacy of vaccines was assessed.
By the beginning of June 2021, almost 11% of the world's population had received at least one dose of a coronavirus disease 2019 (COVID‐19) vaccine. 1 This represents an extraordinary scientific and logistic achievement — in 18 months, researchers, manufacturers and governments collaborated to produce and distribute vaccines that appear ...
Real-world studies may answer key questions about immunizations for RSV that could not be fully addressed in prelicensure trials, including (1) the durability of protection from RSV immunization; (2) whether effectiveness varies by vaccine product in older adults, strategy (maternal vaccination or infant immunization) in young infants, and risk ...
The Coronavirus Efficacy (COVE) phase 3 trial was launched in late July 2020 to assess the safety and efficacy of the mRNA-1273 vaccine in preventing SARS-CoV-2 infection. An independent data and ...
6 Clinical Excellence Research Center, School of Medicine, Stanford University, CA, USA. Electronic address: [email protected]. ... Results: Pfizer and Moderna mRNA COVID-19 vaccines were associated with an excess risk of serious adverse events of special interest of 10.1 and 15.1 per 10,000 vaccinated over placebo baselines of 17.6 and ...
Introduction "The impact of vaccination on the health of the world's peoples is hard to exaggerate. With the exception of safe water, no other modality has had such a major effect on mortality reduction and population growth" (Plotkin and Mortimer, 1988).The development of safe and efficacious vaccination against diseases that cause substantial morbidity and mortality has been one of the ...
Vaccination has long been recognized as one of the most effective ways to reduce child mortality. It has played a significant role, particularly for children, and is considered a major achievement and relevant in preventing childhood diseases worldwide. This study looks at the uptake and determinants of childhood vaccination status among children under the age of one year, for Gambia, Sierra ...
As mass vaccination campaigns against coronavirus disease 2019 (Covid-19) commence worldwide, vaccine effectiveness needs to be assessed for a range of outcomes across diverse populations in a nonc...
Introduction. Childhood vaccines save an estimated 2-3 million lives worldwide every year, which has contributed substantially to the reduction in global infant mortality rate from 65 per 1,000 live births in 1990 to 29 in 2018. 1, 2 Vaccines are found to be the most cost-effective approach for reducing childhood disease burden, especially when compared with interventions such as clean water ...
Process of developing the consensus statement. The consultation process of coming to agreement on a Consensus Statement included setting up a time-limited Working Group of staff of organizations working in vaccine delivery costing (who are also the authors of this paper), conducting a review of guidance documents, costing tools, and other documents and the costing terms, methods, and ...
Adding appropriate adjuvants can decrease the required dosage of antigens, reduce the number of vaccinations needed, and broaden immune protection 95; adjuvant research is an important direction ...
Slightly more than half the participants (56.9%) had received previous Covid-19 vaccination (most commonly with mRNA vaccines), and 49.9% had at least one risk factor for severe Covid-19; the most ...
Vaccines not only afford the best protection against infectious disease but can serve as strong deterrence factors as well. From a bioterrorist perspective, vaccine-resistant agents are more difficult to engineer than drug-resistant agents. But the potential market has been too small and uncertain to encourage the vaccine industry to make large investments in research, development, and ...