
An official website of the United States government
The .gov means it’s official. Federal government websites often end in .gov or .mil. Before sharing sensitive information, make sure you’re on a federal government site.
The site is secure. The https:// ensures that you are connecting to the official website and that any information you provide is encrypted and transmitted securely.
- Publications
- Account settings
Preview improvements coming to the PMC website in October 2024. Learn More or Try it out now .
- Advanced Search
- Journal List
- Ther Adv Vaccines Immunother


Comprehensive literature review on COVID-19 vaccines and role of SARS-CoV-2 variants in the pandemic
Charles yap.
School of Medicine, National University of Ireland, Galway, Ireland
Abulhassan Ali
Amogh prabhakar, akul prabhakar, ying yi lim, pramath kakodkar.
School of Medicine, National University of Ireland, Galway, University Road, Galway H91 TK33, Ireland
Since the outbreak of the COVID-19 pandemic, there has been a rapid expansion in vaccine research focusing on exploiting the novel discoveries on the pathophysiology, genomics, and molecular biology of the severe acute respiratory syndrome coronavirus 2 (SARS-CoV-2) infection. Although the current preventive measures are primarily socially distancing by maintaining a 1 m distance, it is supplemented using facial masks and other personal hygiene measures. However, the induction of vaccines as primary prevention is crucial to eradicating the disease to attempt restoration to normalcy. This literature review aims to describe the physiology of the vaccines and how the spike protein is used as a target to elicit an antibody-dependent immune response in humans. Furthermore, the overview, dosing strategies, efficacy, and side effects will be discussed for the notable vaccines: BioNTech/Pfizer, Moderna, AstraZeneca, Janssen, Gamaleya, and SinoVac. In addition, the development of other prominent COVID-19 vaccines will be highlighted alongside the sustainability of the vaccine-mediated immune response and current contraindications. As the research is rapidly expanding, we have looked at the association between pregnancy and COVID-19 vaccinations, in addition to the current reviews on the mixing of vaccines. Finally, the prominent emerging variants of concern are described, and the efficacy of the notable vaccines toward these variants has been summarized.
Introduction
The coronavirus disease 2019 (COVID-19) pandemic caused by the severe acute respiratory syndrome coronavirus 2 (SARS-CoV-2) has resulted in over 192 million cases and 4.1 million deaths as of July 22, 2021. 1 This pandemic has brought along a massive burden in morbidity and mortality in the healthcare systems. Despite the implementation of stringent public health measures, there have been devasting effects in other sectors contributing to our economy. This has plunged the global economies toward deep recession and has racked up a debt of approximately 19.5 trillion USD. 2
Immune protection in COVID-19 infection can be conceptualized as a spectrum wherein sterile immunity is at the end of positive spectrum. This is followed by transient infection (<3 days) and asymptomatic infection (~1 week). The negative spectrum of immune protection includes patients who are symptomatic, or hospitalized, or admitted to the intensive care unit for multiorgan support. The extreme end of the negative spectrum of immune protection is encompassed by case fatality. The vaccine will intervene prior to the viral insult and stabilize the population at the positive end of the spectrum of the immune protection. It will also prevent the perpetuating cycle of infection and reinfection via variants of SARS-CoV-2 virus in those who have achieved prior convalescence. One study by Dan et al. showed that in patients infected with COVID-19, immunological memory to SARS-CoV-2 remained intact for up to 6 months. 3 Unfortunately, there is no long-term data on the duration of protected immunity against SARS-CoV-2 in patients after convalescence. Therefore, these patients may also require vaccination but the current priority for vaccination can be stretched relative to the unaffected population.
While the ideal goal of the COVID-19 vaccine roll-out is to instill a global herd immunity; it is important to remember that this goal may never be reached. Furthermore, additional goals of vaccination may be to reduce mortality and stress on healthcare systems by reducing the cases of admitted patients. Various countries have already approved COVID-19 vaccines for human use, and more are expected to be licensed in the upcoming year. It is important that these vaccines are safe, efficacious, and can be deployed on a large scale. It is also prudent to eliminate the concerns of both the scientific and general community regarding its effectiveness, side-effects, and dosing strategies.
Historically, the process of vaccine manufacturing and clinical trials required approximately 10 years, but due to the burden of this disease, various observational studies were expedited so that all crucial information regarding the vaccine pharmacokinetics, pharmacodynamics, dosing, efficacy, and adverse events can be collected within a short period of time. Furthermore, there is a need to provide a compilation of accredited and appraised scientific literature on each of these approved vaccines with an aim to instill public health knowledge and vaccine literacy to members of the scientific and general community. A section dedicated to COVID-19 vaccines and pregnancy is also included in the penultimate section of this review.
Finally, the emergence of the SARS-CoV-2 viral variants of concern (VOC) has attained increased replication, transmission, and infectivity warranting exploration of these genomic mutations as their phenotypes. Hence, the final section of this review will aim to clarify the jargon, highlight the vaccine efficacy (VE) against VOCs, and eliminate any misinformation regarding these variants.
Vaccine physiology
The global burden of the pandemic requires an efficacious vaccine that elicits a lasting protective immune response against SARS-CoV-2. This will be an essential armament for the prevention and mitigation of the downstream morbidity and mortality caused by SARS-CoV-2 infection. As of July 20, 2021, there are approximately 108 vaccines in clinical development and 184 vaccines in pre-clinical development with several vaccines being distributed globally. 4
The technologies employed in the vaccine synthesis and development aim to trigger the adaptive immune system and elicit memory cells that will protect the body from subsequent infections. These technologies may be mRNA-based vaccines such as the Moderna and Pfizer/BioNTech, inactivated virus vector vaccines, DNA vaccines, and numerous other technologies. 5
Due to the urgent implementation of vaccine development, the most obvious target will be the robust proteins expressed on the surface of the virus. Therefore, these technologies target molecular expression of the trimeric SARS-CoV-2 spike (S) glycoprotein. These targets could include its mRNA, DNA, full S1 subunit, or fusion subunits. The S protein is a major component of the virus envelope, it is vital for viral fusion, receptor binding, and virus-entry through recognition of host-cellular receptor. The S protein comprises of two main functional units, the S1 subunit, which contains the receptor-binding domain (RBD) and the S2 subunit which is responsible for virus fusion with the host-cell membrane. 6 The choice to proceed with S protein as the target was reinforced when a study by Dan et al. confirmed that in 169 patients infected with SARS-CoV-2, spike-specific immunoglobulin G (IgG) remained stable for over 6 months. 3 In addition, both spike-specific CD4+ T-cells (CD137+ and OX40+) and spike-specific CD8+ T-cells (CD69+ and CD137+) were present at the 6-month post-convalescence period, but their subpopulations exhibited a steady decline with a half-life of 139 days and 225 days, respectively. 3
There are subtle differences in the mechanism by which the different vaccine products interact within host cells to induce immunity. Many successful vaccines of the 20 century utilized the target proteins directly such as the tetanus and pertussis vaccine. A summary of the major types of vaccines and their mechanism of action are shown in Figure 1 .

Summary of major vaccine types and their mechanism of action.
DNA, deoxyribonucleic acid; HPV, Human papillomavirus; mRNA, messenger ribonucleic acid; MMR, Measles, Mumps, and Rubella; SARS-CoV-2, severe acute respiratory syndrome coronavirus 2.
Historically, vaccines usually contained adjuvants which are protein sensitizers that heighten the migratory and sampling response of antigen presenting cells (APCs). Interestingly, the current mRNA vaccines are engineered to code for their own sensitizing protein alongside the S-protein epitopes. Therefore, these new mRNA vaccines usually do not contain any adjuvants. In addition, the mRNA vaccines utilize lipid nanoparticles to deliver the genetic material of a viral S-protein. Contrastingly, vaccines such as the AstraZeneca vaccine may employ a chimpanzee adenovirus vector to carry the DNA genome of the S-protein to the host-cell. 7 Once undergoing the processes of transcription and translation into proteins, these are trafficked and expressed on the host cell surface wherein the adaptive immune system mounts a response via the major histocompatibility complex (MHC) molecules ( Figure 2 ).

Mechanism of induction of immunity through vaccination.
APC, antigen presenting cells; DNA, deoxyribonucleic acid; MHC, major histocompatibility complex; mRNA, messenger ribonucleic acid; SARS-CoV-2, severe acute respiratory syndrome coronavirus 2.
There are two types of MHC molecules, the first one that will be discussed is the MHC-II, which is found exclusively on APC: these comprise of B-cells, macrophages, and dendritic cells in the lymph nodes. Once the S-protein antigen is presented at the cell surface of the MHC-II molecules, the naïve helper T-cell’s (Th Cells) T-cell receptor (TCR) complex will interact with this antigen leading to activation of CD4+ Th cells. This activation is perpetuated by a secondary activation signal with B7 on the APC recognizing the CD28 on the Th cell which triggers the proliferation of Th cells that can recognize the S-protein antigen. Activated CD4+ Th cells then secrete numerous cytokines, namely interleukin (IL)-2 which activates CD8+ cytotoxic T-cells (Tc cell) and trigger clonal expansion of B-cells in memory B-cells and plasma cells. The cytokines IL-4 and IL-5 facilitate B-cell isotype switching and maturation to plasma cells; promoting secretion of IgG antibodies against S-protein. 8 Formation of antibodies allows the immune system to direct an immune response against cells expressing the S-protein of the virus. The second process involves MHC-I, which activates CD8+ Naïve Tc cells through TCR complex interaction with processed endogenously synthesized S-protein expressed on MHC-I. MHC-I is expressed in all nucleated cells, APCs, and platelets and require a second activation signal provided by IL-2 from activated CD4+ Th cells. This activates CD8+ Tc cells which can mount a cytotoxic response against SARS-CoV-2-infected cells through two mechanisms of apoptosis. The first mechanism is the secretion of perforin which create pores to allow granzyme to enter the targeted cell, thus activating apoptosis. The second mechanism is via the expression of FasL, which binds Fas on target cells and induces apoptosis. 8 A crucial part of this process is the stimulation of memory T-cells and memory B-cells. Importantly, while the SARS-CoV-2 vaccine’s lasting effect is still being researched in the context of the pandemic, theoretically these should provide lasting immunity and allow the immune system to mount a faster and more effective response should a vaccinated individual encounter the virus in the future.
Current prominent COVID-19 vaccines
Biontech/pfizer.
The BNT162b2 COVID-19 vaccine developed by BioNTech and Pfizer is a lipid nanoparticle-formulated, nucleoside-modified RNA vaccine that encodes a prefusion membrane-anchored SARS-CoV-2 full-length spike protein. 9 It was the first vaccine approved by the US Food and Drug Association (FDA) and now it has been approved in many other countries. 10 The BNT162b2 COVID-19 vaccine may be stored at standard refrigerator temperatures prior to use, but it requires very cold temperatures for long-term storage and shipping (−70°C) to maintain the stability of the lipid nanoparticle. In a phase-1 trial, it was compared to another vaccine candidate BNT162b1, and it was found to have a milder systemic side-effect profile with a similar antibody response. 11 Therefore, it was pushed forward to a blinded phase-2/3 clinical study. 9 In total, 43,548 participants were randomized to receive either two doses of the BNT162b2 vaccine (n = 21,720) or a placebo (n = 21,728) 21 days apart. The participant ages ranged from 16 to 91 years, 35.1% of participants were classified as having obesity and comorbidities within participants included HIV, malignancy, diabetes, and vascular diseases. 9 Based on the results of the study, 7 days after the second BNT162b2 dose, the VE was 95% (95% confidence interval (CI), 90.3–97.6) with only eight observed cases of COVID-19 in the vaccine recipients and 162 cases in the placebo recipients. 9 The efficacy remained consistent across subgroups characterized by age, sex, race, ethnicity, body mass index (BMI), and comorbidities (generally 90–100%). 9 Although there were 10 cases of severe COVID-19 with onset after the first dose, only one occurred in a vaccine recipient and nine in placebo recipients. Like the phase-1 trial results, the safety profile remained favorable with the most common local reaction being mild-to-moderate pain at the injection site while the most common systemic symptoms were fatigue and headache (reported in ⩾50%). 9 In both the vaccine and placebo group, the incidence of severe adverse events did not differ significantly (0.6% and 0.5%, respectively) and no deaths occurred related to the vaccine. As indicated by the manufacturer’s information, contraindications for use include hypersensitivity to the active substance or any of the excipients. 12 These studies show that the mRNA-vaccine BNT162b2 is safe and effective in protecting against COVID-19. However, further investigations are needed to confirm long-term safety and to establish safety and efficacy for populations not included in this study.
The mRNA-1273 vaccine, developed by Moderna, relies on mRNA technology to encode prefusion stabilized SARS-CoV-2 spike protein. It is the second COVID-19 vaccine to receive emergency use approval by the US FDA, and it is given as two 100-µg doses intramuscularly into the deltoid muscle, 28 days apart. 13 Storage of the vaccine is done at temperatures between −25°C to −15°C for long-term storage, 2°C to 8°C for 30 days, or 8°C to 25°C for up to 12 hours. Results from the COVE phase-3 trial showed that the mRNA-1273 vaccine was effective at preventing COVID-19 illness in persons 18 years of age or older. A total of 30,420 participants aged 18 years or older were randomized 1:1 to receive either two doses of the vaccine or a placebo, 28 days apart. 14 The mean age of the participants was 51.4 years, and enrollment was adjusted for equal representation of racial and ethnic minorities. In the trial, symptomatic COVID-19 illness occurred in 11 participants within the vaccine group versus 185 participants within the placebo group, showing a 94.1% (95% CI, 89.3–96.8%) efficacy of the vaccine. Efficacy was similar across age, sex, race, and ethnicity as well as in patients with and without risk factors for severe disease (e.g. chronic lung disease, cardiac disease, and severe obesity). Importantly, a secondary endpoint for determining the efficacy of the vaccine in preventing severe COVID-19 was also used. All 30 participants with severe COVID-19 were in the placebo group, indicating a 100% efficacy of no hospital admissions. 14 Regarding the side effects of the vaccine, adverse events at the injection site and systemic adverse events occurred more commonly with the mRNA-1273 group compared to the placebo. The most common local reaction was mild to moderate pain at the injection site (75%). The most common systemic symptoms were fatigue, myalgia, arthralgia, and headache (50%). 14 The overall incidence of serious adverse events did not differ significantly between groups and no deaths occurred in relation to the vaccine. While this vaccine is already being administered, further investigations are still necessary to establish safety and efficacy profiles for populations not included in this study as well as to assess its long-term effects. Current contraindications of the mRNA-1273 vaccine include any persons with known allergy to polyethylene glycol (PEG), another mRNA vaccine component or polysorbate. 15
AstraZeneca
The Oxford and AstraZeneca ChAdOx1 COVID-19 vaccine uses a chimpanzee adenovirus vector to deliver the genetic sequence of a full-length spike protein of SARS-CoV-2 into host cells. 16 The storage for the ChAdOx1 vaccine is favorable, as it may be refrigerated at 2°C–8°C for 6 months. Pooled analysis of four ongoing clinical studies was used to assess efficacy, safety, and immunogenicity of the ChAdOx1 vaccine: COV001 (phase 1/2), COV002 (phase 2/3), COV003 (phase 3), and COV005 (phase 1/2). 17 Across the four studies participants over 18 were randomized to receive either the vaccine or a control (meningococcal group A, C, W, or saline). ChAdOx1 vaccine recipients received two standard doses (SDs) of the vaccine (SD/SD cohort) except for a subset in the COV002 trial who received a half lower dose (LD) followed by an SD (LD/SD cohort). 17 In the four studies, there was a total 23,848 participants, all of whom were used for gathering safety data; only 11,636 participants from the COV002 and COV003 trials were included in the primary efficacy analysis. 17 Of the 11,636 participants in the efficacy analysis, 2741 were in the LD/SD cohort, 88% were between 18 and 55 years old, and comorbidities present included cardiovascular disease, respiratory disease, and diabetes. 17 The results show that in the intended dosing regimen (SD/SD cohort), the VE was 62.1% (95% CI, 41.0–75.7) ⩾14 days after the second injection for symptomatic COVID-19 (27 cases vs 71 cases respectively). 17 In the group that received an LD (LD/SD cohort), the VE was 90.0% (95% CI, 67.4–97.0; 3 cases vs 30 cases, respectively) while across the two dosing regimens the overall efficacy was 70.4% (95.8% CI, 54.8–80.6;30 cases vs 101 cases, respectively). 17 The higher efficacy observed in the LD/SD cohort can be attributed to this group having a longer dosing interval between the two doses in comparison to the SD/SD cohort. Regarding safety, most of the adverse events were mild-moderate with the most frequently reported being injection site pain/tenderness, fatigue, headache, malaise, and myalgia. 18 About 175 serious adverse events were noted, only three of which were possibly linked to intervention: transverse myelitis 14 days after second dose, haemolytic anemia in a control recipient and fever >40°C in a participant still masked to group allocation. One contraindication for use of the vaccine is hypersensitivity to any of its components. In very rare cases, AstraZeneca has been associated internationally with venous thromboembolic events with thrombocytopenia with current estimates being 10–15 cases per million vaccinated patients. 19 This adverse event has been termed thrombosis with thrombocytopenia syndrome (TTS). In summary, these studies demonstrate that the AstraZeneca ChAdOx1 vaccine has a good efficacy and side-effect profile. Limitations include that less than 4% of participants were >70, no one over 55 got the mixed-dose regimen (LD/SD cohort), and those with comorbidities were a minority. Additional investigations are required to analyze long-term effects and assess efficacy and safety in populations not included or underrepresented.
Janssen COVID-19 vaccine
The Janssen (Johnson & Johnson) COVID-19 vaccine, developed by Janssen Pharmaceutical in Netherlands. It is a single-dose intramuscular (IM) vaccine that contains a recombinant, replication incompetent human adenovirus (Ad26) vector encoding the spike protein of SARS-CoV-2 in the stabilized conformation. 20 It can be stored between 2°C and 8°C for up to 6 hours or at room temperature for a duration of 2 hours. The ENSEMBLE Phase-3 trial (n = 43,783) is a randomized, double-blind, placebo-controlled study which included participants ⩾18 years. Efficacy assessment was performed at day 14 and 28. The primary outcome only included moderate and severe (hospitalization and death) infection. Overall, the VE in the moderate to severe cohort was 66.9% (95% CI: 59.0–73.4) at 14 days and 66.1% (95% CI: 55.0–74.8) at 28 days. 20 In the severe cohort, the VE was 76.7% (95% CI: 54.6–89.1) and 85.4% (95% CI: 54.2–96.9) at day 14 and 28 days, respectively. 20 At the time of the study, 96.4% of the strains in the United States, 96.4% were identified as the Wuhan-H1 variant D614G. The VE in the United States for the moderate to severe cohort was 74.4% (95% CI: 65.0–81.6) and 72.0% (95% CI: 58.2–81.7) at 14 days and 28 days, respectively. 20 In the US severe cohort, the VE was 78.0% (95% CI: 33.1–94.6) and 85.9% (95% CI: −9.4 to 99.7) at day 14 and 28 days, respectively. 20 Alternatively, 94.5% of the strains in South Africa were identified as beta variant. The VE in South Africa for the moderate to severe cohort was 52.0% (95% CI: 30.3–67.4) and 64.0% (95% CI: 41.2–78.7) at 14 days and 28 days, respectively. 20 In the South African severe cohort, the VE was 73.1% (95% CI: 40.0–89.4) and 81.7% (95% CI: 46.2–95.4) at day 14 and 28 days, respectively. 20 In Brazil, 69.4% of the strains were identified as P.2 lineage variant and 30.6% were identified as Wuhan-H1 variant D614G. The VE in Brazil for the moderate to severe cohort was 66.2% (95% CI: 51.0–77.1) and 68.1% (95% CI: 48.8–80.7) at 14 days and 28 days, respectively. 20 In the Brazilian severe cohort, the VE was 81.9% (95% CI: 17.0–98.1) and 87.6% (95% CI: 7.8–99.7) at day 14 and 28 days, respectively. 20 The most common localized solitary adverse reaction was the injection site pain (48.6%). Conversely, the most common systemic adverse reactions included headache, fatigue, myalgia, and nausea. 20 In the post authorization phase, adverse reaction included anaphylaxis, thrombosis with thrombocytopenia, Guillain Barré syndrome, and capillary leak syndrome. 20 Overall, the data demonstrate that the Janssen vaccine has a good efficacy and side-effect profile.
Sputnik V or Gam-COVID-Vac, developed by the Gamaleya Institute, is a recombinant human adenovirus-based vaccine that uses two different vectors (rAd26 and rAd5) to carry the gene encoding for the spike protein of SARS-CoV-2. Only one vector (rAd26) is given at dose 1 and the other (rAd5) at dose 2. This strategy prevents immunity against the vector. It can be stored as either a liquid at −18°C, or it can be freeze-dried and stored at 2°C to 8°C. 21 Regarding the safety and efficacy of the vaccine, both were evaluated in a randomized, double-blind phase-3 trial performed in Moscow, Russia. In the trial, a total of 21,977 participants aged 18 years or older were randomized in a 3:1 ratio to the vaccine or placebo groups. Two doses of the vaccine or placebo were given 21 days apart to the respective groups. 21 The mean age of the participants was 45.3 years, and the majority of participants were Caucasian (98.5%). 21 From 21 days after the first dose of the vaccine, efficacy against symptomatic COVID-19 illness was 91.6% (95% CI, 85.6–95.2%) with 16 confirmed cases of COVID-19 in the vaccine group and 62 confirmed in the placebo group. 21 There were also 20 cases of moderate to severe COVID-19 infection confirmed in the placebo group at least 21 days after the first dose and 0 in the vaccine group, indicating a VE of 100% against moderate to severe infection. 21 The most common adverse effects in both groups were flu-like illness, injection site reactions, headaches, and asthenia, with the majority being grade 1 (94.0%). 21 Serious adverse events were also reported in both the vaccine group and placebo group, but they were deemed not to be associated with the vaccination. Further investigations are still needed to determine the duration of protection of the vaccine and to determine the safety and efficacy of the vaccine in populations not included in the study (e.g. children, adolescents, and pregnant and lactating women).
CoronaVac is an inactivated vaccine developed by SinoVac Biotech containing inactivated SARS-CoV-2. 22 The vaccine can be stored at 2°C to 8°C for up to 3 years making it an attractive option for development. Two phase-1/2 clinical trials assessed the safety, tolerability, and immunogenicity of the CoronaVac vaccine. 22 , 23 The first study (18–59 years old included only) placed 744 participants in either a vaccine or placebo group where they were further divided based on vaccination schedule and dosage (3 and 6 μg). In the second study (⩾60 years old included only), 422 participants were randomized to receive two doses of CoronaVac or placebo 28 days apart and then further divided based on dosage amount only (3 and 6 μg for phase 1; 1.5, 3, and 6 μg for phase 2). Safety results from both trials show a favorable side-effect profile with most symptoms being transient and of mild severity. The most common adverse effect was injection site pain; others included fatigue and fever. In the 18–59 years old study, one serious adverse event of acute hypersensitivity was possibly related to vaccination. 22 No serious adverse events were associated with the vaccine or placebo in the ⩾60-year-old study. Between the dosage amounts in both studies, the tolerability was consistent and the immunogenicity was also similar for the 3 and 6 μg doses (less in 1.5 μg). 23 Multiple phase-3 trials have also taken place to determine the effectiveness of CoronaVac in countries, such as Brazil, Indonesia, and Turkey. In the Brazil trial, 252 cases of COVID-19 were recorded from roughly 9200 health care workers, with 167 in the placebo group and 85 in the vaccine group. 24 The reported efficacy of the vaccine in preventing mild and severe COVID-19 infection was 50.4%. In comparison, the Turkey trial reported that the vaccine was 83.5% effective at preventing symptomatic infection based on 29 COVID-19 cases among 1,322 volunteers while results from the Indonesia trial found that the vaccine was 65.3% effective at preventing symptomatic infection based on 25 COVID-19 cases among 1,600 people. 24 Some reasons for the lower efficacy of CoronaVac in the Brazil trial may include increased likelihood of exposure to the virus since participants were healthcare workers, and insufficient time for participants to reach peak immunity since the doses were administered only 2 weeks apart. 24 The phase-3 SinoVac study in Chile showed the VE 14 days post second dose to prevent symptomatic COVID-19 (67%, 95% CI: 65–69%), hospital admission (85%, 95% CI: 83–87%), intensive care unit (ICU) admission (89%, 95%CI: 84–92%) and death (80%, 95%CI: 73–86%). 25 The Phase-3 SinoVac trial in Brazil showed an overall VE against symptomatic COVID-19 (50.7%, 95% CI: 35.9–62%), moderate cases requiring hospitalization (83.7%, 95% CI: 58–93.7%), and severe cases requiring hospitalization (100%, 95%CI: 56.4–100%). 26 As with any vaccine, a contraindication for CoronaVac is anaphylaxis to it or to one of its constituents.
Other prominent COVID-19 vaccines
Due to the disease burden of SARS-CoV-2, the development and manufacturing of COVID-19 vaccines has been occurring at a remarkable pace which has not been seen before. There are many emerging vaccines with different mechanisms of actions that will be briefly explored. Bharat Biotech, an Indian company, has designed the inactivated COVID-19 vaccine Covaxin (BBV152). Once inside the body, the inactivated viruses can initiate an immune response through the interaction of surface proteins with APCs. Phase-1/2 trials showed no serious side effects and phase-3 trials are currently underway. 27 The state-owned Chinese company Sinopharm has also made an inactivated COVID-19 vaccine called BBIBP-CorV. The Sinopharm phase-3 trial showed that the VE in symptomatic cases for the WIV04 strain-based vaccine (72.8, 95% CI: 58.1–82.4%) and HB02 strain-based vaccine (78.1 95% CI: 64.8–86.3%). 28 , 29 It is approved in Bahrain, U.A.E, and China. NVX-CoV2373 is another promising vaccine produced by Novavax. It is a protein subunit vaccine made by assembling SARS-CoV-2 spike proteins into nanoparticles. A phase-3 trial in the United Kingdom displayed an efficacy rate of 89.3%; however, a phase-2 trial in South Africa had an efficacy just under 50%. 28 This discrepancy is thought to arise because of a new variant in South Africa. Other emerging vaccines include CoVLP produced by Medicago which uses the plant N. benthamiana to create virus-like particles that mimic SARS-CoV-2, CVnCoV produced by CureVac which is an mRNA vaccine, Convidecia produced by CanSino Biologics which is adenovirus based (Ad5), Ad26.COV2.S produced by Johnson & Johnson which is also adenovirus based (Ad26), and ZF2001 created by Anhui Zhifei Longcom which is a protein subunit vaccine. Even though highly effective, COVID-19 vaccines are already in use, it is still important to have a range of vaccines such as those listed above to bring the pandemic under control. Having a diverse profile ensures that vaccines will work for individuals from all ethnic backgrounds and with various underlying health conditions. 30 Getting the virus under control will also require doses for a large proportion of the world. To meet this requirement as soon as possible, having multiple vaccines will help in maximizing the volume of doses that can be produced. In addition, there are many technical issues such as cold storage and transportation, cost, and dosing of certain vaccines that arise when trying to vaccinate remote populations. For example, both the Pfizer-BioNTech and Moderna vaccines are expensive and transported at temperatures of −70°C and −20°C making it difficult to access many locations all at once. Since most vaccines require two doses spaced a few weeks apart, it can be challenging for individuals without regular access to healthcare as well. 30 Such considerations highlight the importance of having a range of single-dose vaccines and vaccines without the need for cold storage. A summary of efficacy, prominent side effects and storage recommendations for all the notable COVID-19 vaccines are shown in Table 1 .
Summary of vaccine efficacy, dosing strategy, and side-effects of different COVID-19 vaccines.
CI, confidence interval; COVID-19, coronavirus disease 2019; IM, intramuscular.
Post-vaccination contagion
With the endurance of the COVID-19 vaccine still being heavily researched, a chief concern is the sustainability of the vaccine-mediated immune response. This is important in the consideration of whether vaccinated individuals could still contract, transmit, or be carriers of SARS-CoV-2 virus. Vaccinated individuals currently may not understand the rationale behind why social restriction rules still apply to them. Most COVID-19 mRNA vaccines require at least 3 weeks to mount an immunological response and create the required antibodies and proliferate accessory cells of the adaptive immune system of the appropriate recognition repertoire. 50 This may be particularly relevant in the context of travel, as the World Health Organization (WHO) states that a proof of vaccination should not exempt international travelers from complying with social restrictions and risk-reduction measures. 51
Contraindications for COVID-19 vaccines
All vaccines are contraindicated in cases of documented hypersensitivity to the active substance or any of the excipients. There are a set of general guidelines relative to patients which must be adhered to until further information is provided; predominantly regarding groups such as pregnant or lactating women and immunodeficient patients. The Centers for Disease Control and Prevention (CDC) considers absolute contraindications to patients who have had severe anaphylactic reactions to a previous dose of an mRNA COVID-19 vaccine or PEG, a component of the vaccine. Moreover, immediate allergic reactions of any severity to polysorbate are also a significant contraindication. Importantly, there are many precautions which are not classified as contraindications but must be considered, such as patients who have had allergic reactions to any vaccine or injectable therapy. In the cases of patients with a precaution to the vaccine, they should be counseled on the benefits and risks, but are not contraindicated from vaccination. 15 In the instance of patients with autoimmune diseases, there is currently insubstantial data regarding the efficacy of the vaccine; however, current guidelines suggest that individuals with autoimmune conditions may take the vaccine if they do not have any absolute contraindications. In the case of patients with HIV, limited data from COVID-19 mRNA vaccination trials suggest that they can receive the vaccine barring any contraindications.
COVID-19 vaccines and pregnancy
Prior to discussing the relationship between the current vaccines for COVID-19 and pregnancy, it is crucial to gain an insight of the relationship between pregnancy and COVID-19 itself. Adhikari et al. showed that there was no difference in the frequency of Caesarean section, pre-eclampsia, preterm births, and abnormal fetal cardiotocography in pregnant women with and without SARS-CoV-2 infection. In addition, examination of the placenta revealed were no abnormalities, which were initially suspected due to the cross-matching between the SARS-CoV-2 spike protein and the placental synctyin-1 protein. 52 Similarly, there was no association found between COVID-19 and first-trimester spontaneous abortions. 53 A systematic review and meta-analysis revealed that COVID-19 leads to higher preterm deliveries (odds ratio (OR): 3.01, 95% CI: 1.16–7.85) and an increase in the ICU admission rates (OR: 71.63, 95% CI: 9.81–523.06) in pregnant women. 54
Pregnancy remained an exclusion criterion for all the COVID-19 vaccine trial; therefore, the efficacy of the COVID-19 vaccines in pregnant women is unavailable. However, given the effectiveness of the influenza vaccines elucidated in a meta-analysis conducted by Quach et al ., it can be hypothesized that the effects of pregnancy on the vaccine would be minimal, but more data would be needed for confirmation. 55 Pfizer’s animal studies revealed antibodies in the maternal rats, fetus, and offspring, in addition to no effects on fertility pregnancy or fetal development. 56 A similar study was conducted with the Moderna vaccine which led the US FDA to conclude that the vaccine did not have any adverse effects on female reproduction, fetal development, or postnatal development. 34 Furthermore, the Oxford-AstraZeneca vaccine animal studies are still pending. However, as a precaution, the National Immunization Advisory Committee (NIAC) has recommended for the two-dose schedule to not commence before 14 weeks of gestation and to be completed by week 33 of gestation. This precaution reduces any potential associations with miscarriage or pre-term birth. 57
Despite the exclusion of pregnancy in the preliminary stages of the trials, 23 Pfizer, 13 Moderna, and 21 AstraZeneca subjects became pregnant after enrolment into the trial. Among this cohort, there was one miscarriage part of the Pfizer control group, no miscarriages part of the Pfizer vaccine group, one miscarriage part of the Moderna control group, no miscarriages part of the Moderna vaccine group, three miscarriages part of the AstraZeneca control group, and two miscarriages part of the AstraZeneca vaccine group. While these preliminary numbers support the current guidelines regarding the vaccines being safe in pregnancy, it is crucial to be aware of the ongoing studies as new data emerges.
The CDC v-safe COVID-19 Pregnancy Study explored the effect of mRNA vaccine (Pfizer-BioNTech or Moderna) on the pregnancy. The pregnancy loss within those with a completed pregnancy included a spontaneous abortion (<20 weeks) rate of 12.6% (104 out of 827) and stillbirth (⩾20 weeks) incidence of 0.1% (1 out of 725). 58 The neonatal outcomes within the live birth infant cohort showed preterm birth (<37 weeks) incidence at 9.4% (60 out of 636), small for gestational age incidence of 3.2% (23 out of 724), and congenital anomalies were seen in 2.2% (16 out of 724). 58 No neonatal deaths were observed in this study.
Vaccine dosing strategies
Limited vaccine resources have caused some governments to extend the date of the second dose beyond the recommended manufacturer date. On December 30, NHS England had made the decision to prioritize the administration of the first doses, and to extend the second doses of the vaccine to the end of 12 weeks, rather than the recommended 3–4 weeks as shown in the clinical phase-3 trial. Pfizer-BioNTech at the time had no data to support this decision, and thus stated that the safety and efficacy of the vaccine had not been evaluated on different dosing schedules, and importantly, the second dose should not be administered later than 42 days. 59
Newly accrued evidence might warrant changes in the landscape of this vaccination program. Estimation of the effectiveness of the Pfizer-BioNTech after a single dose from the primary data from Israeli population (n = 500,000) showed that from day 0 to day 8 post–vaccination, the likelihood of contracting COVID-19 infection doubled. 60 This result may appear counterintuitive, but it takes 3 weeks for the vaccine to instill efficacy during which this real-world population could have not maintained the stringent public health measures which lead to the increased incidence in COVID-19 in this time-period. Then from day 8 to day 21 the incidence of COVID-19 declined and at day 21 the vaccine effectiveness was documented at 91%. 60 This efficacy was seen to stabilize at 90% for the duration of the study (9 weeks), and the authors of this study extrapolate this stability up to 6 months. 60 This concludes that the single dose of Pfizer-BioNTech is highly protective from day 21 onwards and supports the NHS England’s vaccination policy for extending gaps between the doses. The data from the Early Pandemic Evaluation and Enhanced Surveillance of COVID-19 (EAVE II) trial in the Scottish population revealed that a single dose of Pfizer (n = 650,000) and Oxford-AstraZeneca (n = 490,000) vaccines resulted in a decline in hospitalization at 4 weeks by 84% and 94%, respectively. 61
However, the trials for the Oxford-AstraZeneca vaccine included varied spacing schedules between doses. The findings from these trials displayed that a greater space between the first and second dose provided a superior immune response. This is supported by a combined trial between a UK and Brazil study, which demonstrated a higher VE 14 days after a second dose in patients who had greater than 6 weeks between their first and second dose than patients who had less than 6 weeks by 53.4%. 17 , 62
It was also proposed that to meet the supply shortage that vaccine dose can be halved. Half-dose of Moderna vaccine (50ug) was in a phase-IIa trial. Immune response in the half-dose group compared to those that received a full dose were the same. Therefore, this dosing strategy is supported from an immunogenicity perspective. It is reasonable to infer that the immunogenicity would translate to immune protection, but unfortunately no clinical trial has validated the immune protection for this dosing strategy.
SARS-CoV-2 genome mutations
Mutations are changes in the SARS-CoV-2 viral genome that occur naturally over time. These mutations from the parent SARS-CoV-2 virus create variants. A certain amount of genetic variation is expected as SARS-CoV-2 replicates as such it is important to monitor circulating viral variants to collate key mutations. Fortunately, coronaviruses have a slower rate of mutation of 1 to 2 nucleotides per month. 63 These definitions become complicated when environmental factors apply selective pressures on these variants that enable them to express distinct phenotypes that may facilitate viral fitness. This ability of a variant to express distinct phenotypes is termed as a strain. A compilation of beneficial lineage defining mutations can create a strain that has a higher transmission rate or induce severe disease. This raises the question: will the current vaccines or convalescent immunity from a non-variant SARS-CoV-2 infection provide adequate immunological protection against these new variants?
Coronaviruses mutate spontaneously via antigenic drift. This process typically utilizes the virus-specific transcription regulatory network (TRN) sequence to initiate the change, resulting in a new mRNA sequence virus being formed. Homologous and genetic recombination allows for the virus to gain more ecological features and has been speculated to be the reason why SARS-CoV-2 was zoonotic in origin. 64 A variant of the original SARS-CoV-2 virus with a D614G substitution in the spike protein encoding gene emerged in early February 2020, and by June 2020, D614G became the dominant form of the virus circulating globally. 65 Studies have shown that the D614G mutation resulted in increased infectivity and transmissibility. 66 Since then, there have been many viral lineages to note, most notable VOC include the B.1.1.7/20I/501.Y.V1 variant that was first detected in the United Kingdom in October 2020, the B.1.351/20 H/501Y.V2 variant that was detected in South Africa in December 2020, and the Lineage P.1. (B.1.1.28.1) variant that was detected in Tokyo in January 2021 but is believed to have originated from Brazil.
Currently, there exists two open-source real-time software tools to analyze and assign nomenclature of genetic variations in the SARS-CoV-2 virus: Nextstrain and PANGOLIN. 64 , 67 Both refer to the GISAID (Global Initiative on Sharing All Influenza Data) genomic database but have slight differences with regards to their nomenclature to describe various lineages of the virus. The COVID-19 Genomics UK Consortium has also developed CoV-GLUE, an open-source browser application that allows for easy referral of all sequenced SARS-CoV-2 genetic replacements, insertions, and deletions. 68 Therefore, sequencing every local infection will yield a repository to track the development of new mutations and variants.
Notable mutation drivers in the SARS-CoV-2 genome
Before diving deeper into these variants, it is important to understand the physical alteration in the S-protein at a molecular level and the perceived functional advantages that the SARS-CoV-2 gains. Table 2 highlights some of the notable S-protein mutations as they evolve amid the pandemic.
Summary of the physical and functional alterations of S-protein due to notable amino acid substitutions.
RBD, receptor-binding domain; VOC, variants of concern.
Notable emerging VOC
Newly emerged variants of SARS-CoV-2 have now become VOC which can be attributed to their new ability of increased transmission and infectivity. Therefore, it is important to collate the data on the mutations they acquired, the extend of spread, and the efficacy of different vaccines to create a repository for further analysis ( Table 3 ).
Summary of data on features, acquired cluster of S-protein mutations, and vaccine efficacy studies for the major COVID-19 variants of concern.
CI, confidence interval; COVID-19, coronavirus disease 2019; VOC, variants of concern.
There are more variants emerging as the pandemic progresses, but it is important to note that there is still a myriad of available vaccines in our armamentarium that are adequately efficacious in the performed neutralization assays as well as the real-world data. Furthermore, while vaccines induce the antibody-dependent immunity, they can also stimulate other components of the adaptative immune system such as the Memory B-cells, CD8+ Tc cells, and CD4+ Th cells to mount their own response against the viral variants. This can compensate for the reduction in neutralization rate by the vaccine induced antibodies. Interestingly, the adaptative immune system can proliferate libraries of memory B-cells with mutated antibody repertoires that can predict viral variants. Therefore, it is prudent to commence vaccinations in accordance with the local public health bodies. This combined with the continued implementation of public health measures until target level of herd immunity is acquired can lead toward mitigating the prevalence and incidence of COVID-19 variants.
This review highlighted the current available vaccines and candidates being rolled out amid the ongoing prevention measures and summarized the documented findings with regards to their efficacies, side-effects, and storage requirements. An overview of the physiology of immunogenic responses against the disease provided by the more prominent vaccines were discussed, alongside questions regarding the implementation of vaccines; heterologous prime-boosting, vaccine contraindications, dosing strategies, side effects, and the presence of SARS-CoV-2 mutations and variants.
There are still many unanswered questions that need to be addressed with regards to antibodies produced in individuals including their impact on the clinical course and severity of the disease, how long will they remain in the body to protect from the disease, and if what we have is enough to deal with newly emerging variants. Studies on these topics are rapidly being conducted and published on a global scale, and scientific communities are working on the clock to produce as much information to bring us a better understanding on how to deal with this disease.
For this global pandemic to end, it is imperative that people are vaccinated as quickly as possible until herd immunity can be achieved. One aspect of achieving this, in the face of vaccine hesitancy, is to address the lack of community understanding on how vaccines work, the risks, and the factors that keep this area of research volatile and distribution policies ever-changing. In addition, it is important to remain cautious about the information being released and to trust the accredited sources and experts, rather than the aberrant rumors being spread through social media. Nonetheless, the COVID-19 vaccines have shown to be highly promising and we recommend for everyone that is eligible to take the vaccine at the correct dosing interval when they are given the chance as this would potentiate a positive trend toward pandemic resolution.
Authors’ contributions: CY, AA, Amogh P, Akul P, AP performed acquisition and curation of the data; CY, AA, Amogh P, Akul P, AP, YYL and PK analyzed the data, performed interpretation of the data, and wrote of the original draft; YYL and PK performed the critical revision; All authors have read and approved the final manuscript.
Conflict of interest statement: The authors declared no potential conflicts of interest with respect to the research, authorship, and/or publication of this article.
Funding: The authors received no financial support for the research, authorship, and/or publication of this article.

Contributor Information
Charles Yap, School of Medicine, National University of Ireland, Galway, Ireland.
Abulhassan Ali, School of Medicine, National University of Ireland, Galway, Ireland.
Amogh Prabhakar, School of Medicine, National University of Ireland, Galway, Ireland.
Akul Prabhakar, School of Medicine, National University of Ireland, Galway, Ireland.
Aman Pal, School of Medicine, National University of Ireland, Galway, Ireland.
Ying Yi Lim, School of Medicine, National University of Ireland, Galway, Ireland.
Pramath Kakodkar, School of Medicine, National University of Ireland, Galway, University Road, Galway H91 TK33, Ireland.
- Scoping Review
- Open access
- Published: 14 November 2021
Effectiveness and safety of SARS-CoV-2 vaccine in real-world studies: a systematic review and meta-analysis
- Qiao Liu 1 na1 ,
- Chenyuan Qin 1 , 2 na1 ,
- Min Liu 1 &
- Jue Liu ORCID: orcid.org/0000-0002-1938-9365 1 , 2
Infectious Diseases of Poverty volume 10 , Article number: 132 ( 2021 ) Cite this article
57k Accesses
208 Citations
372 Altmetric
Metrics details
To date, coronavirus disease 2019 (COVID-19) becomes increasingly fierce due to the emergence of variants. Rapid herd immunity through vaccination is needed to block the mutation and prevent the emergence of variants that can completely escape the immune surveillance. We aimed to systematically evaluate the effectiveness and safety of COVID-19 vaccines in the real world and to establish a reliable evidence-based basis for the actual protective effect of the COVID-19 vaccines, especially in the ensuing waves of infections dominated by variants.
We searched PubMed, Embase and Web of Science from inception to July 22, 2021. Observational studies that examined the effectiveness and safety of SARS-CoV-2 vaccines among people vaccinated were included. Random-effects or fixed-effects models were used to estimate the pooled vaccine effectiveness (VE) and incidence rate of adverse events after vaccination, and their 95% confidence intervals ( CI ).
A total of 58 studies (32 studies for vaccine effectiveness and 26 studies for vaccine safety) were included. A single dose of vaccines was 41% (95% CI : 28–54%) effective at preventing SARS-CoV-2 infections, 52% (31–73%) for symptomatic COVID-19, 66% (50–81%) for hospitalization, 45% (42–49%) for Intensive Care Unit (ICU) admissions, and 53% (15–91%) for COVID-19-related death; and two doses were 85% (81–89%) effective at preventing SARS-CoV-2 infections, 97% (97–98%) for symptomatic COVID-19, 93% (89–96%) for hospitalization, 96% (93–98%) for ICU admissions, and 95% (92–98%) effective for COVID-19-related death, respectively. The pooled VE was 85% (80–91%) for the prevention of Alpha variant of SARS-CoV-2 infections, 75% (71–79%) for the Beta variant, 54% (35–74%) for the Gamma variant, and 74% (62–85%) for the Delta variant. The overall pooled incidence rate was 1.5% (1.4–1.6%) for adverse events, 0.4 (0.2–0.5) per 10 000 for severe adverse events, and 0.1 (0.1–0.2) per 10 000 for death after vaccination.
Conclusions
SARS-CoV-2 vaccines have reassuring safety and could effectively reduce the death, severe cases, symptomatic cases, and infections resulting from SARS-CoV-2 across the world. In the context of global pandemic and the continuous emergence of SARS-CoV-2 variants, accelerating vaccination and improving vaccination coverage is still the most important and urgent matter, and it is also the final means to end the pandemic.
Graphical Abstract
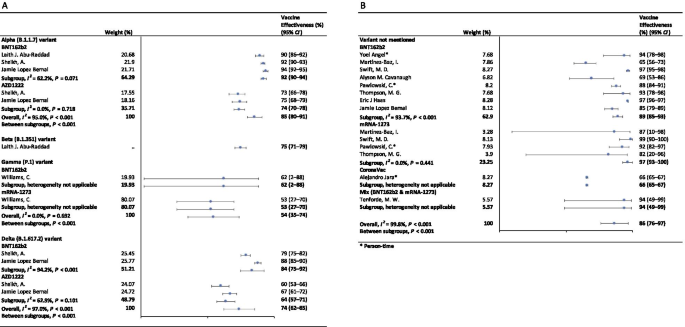
Since its outbreak, coronavirus disease 2019 (COVID-19) has spread rapidly, with a sharp rise in the accumulative number of infections worldwide. As of August 8, 2021, COVID-19 has already killed more than 4.2 million people and more than 203 million people were infected [ 1 ]. Given its alarming-spreading speed and the high cost of completely relying on non-pharmaceutical measures, we urgently need safe and effective vaccines to cover susceptible populations and restore people’s lives into the original [ 2 ].
According to global statistics, as of August 2, 2021, there are 326 candidate vaccines, 103 of which are in clinical trials, and 19 vaccines have been put into normal use, including 8 inactivated vaccines and 5 protein subunit vaccines, 2 RNA vaccines, as well as 4 non-replicating viral vector vaccines [ 3 ]. Our World in Data simultaneously reported that 27.3% of the world population has received at least one dose of a COVID-19 vaccine, and 13.8% is fully vaccinated [ 4 ].
To date, COVID-19 become increasingly fierce due to the emergence of variants [ 5 , 6 , 7 ]. Rapid herd immunity through vaccination is needed to block the mutation and prevent the emergence of variants that can completely escape the immune surveillance [ 6 , 8 ]. Several reviews systematically evaluated the effectiveness and/or safety of the three mainstream vaccines on the market (inactivated virus vaccines, RNA vaccines and viral vector vaccines) based on random clinical trials (RCT) yet [ 9 , 10 , 11 , 12 , 13 ].
In general, RNA vaccines are the most effective, followed by viral vector vaccines and inactivated virus vaccines [ 10 , 11 , 12 , 13 ]. The current safety of COVID-19 vaccines is acceptable for mass vaccination, but long-term monitoring of vaccine safety is needed, especially in older people with underlying conditions [ 9 , 10 , 11 , 12 , 13 ]. Inactivated vaccines had the lowest incidence of adverse events and the safety comparisons between mRNA vaccines and viral vectors were controversial [ 9 , 10 ].
RCTs usually conduct under a very demanding research circumstance, and tend to be highly consistent and limited in terms of population characteristics and experimental conditions. Actually, real-world studies differ significantly from RCTs in terms of study conditions and mass vaccination in real world requires taking into account factors, which are far more complex, such as widely heterogeneous populations, vaccine supply, willingness, medical accessibility, etc. Therefore, the real safety and effectiveness of vaccines turn out to be a major concern of international community. The results of a mass vaccination of CoronaVac in Chile demonstrated a protective effectiveness of 65.9% against the onset of COVID-19 after complete vaccination procedures [ 14 ], while the outcomes of phase 3 trials in Brazil and Turkey were 50.7% and 91.3%, reported on Sinovac’s website [ 14 ]. As for the Delta variant, the British claimed 88% protection after two doses of BNT162b2, compared with 67% for AZD1222 [ 15 ]. What is surprising is that the protection of BNT162b2 against infection in Israel is only 39% [ 16 ]. Several studies reported the effectiveness and safety of the COVID-19 vaccine in the real world recently, but the results remain controversial [ 17 , 18 , 19 , 20 ]. A comprehensive meta-analysis based upon the real-world studies is still in an urgent demand, especially for evaluating the effect of vaccines on variation strains. In the present study, we aimed to systematically evaluate the effectiveness and safety of the COVID-19 vaccine in the real world and to establish a reliable evidence-based basis for the actual protective effect of the COVID-19 vaccines, especially in the ensuing waves of infections dominated by variants.
Search strategy and selection criteria
Our methods were described in detail in our published protocol [PROSPERO (Prospective register of systematic reviews) registration, CRD42021267110]. We searched eligible studies published by 22 July 2021, from three databases including PubMed, Embase and Web of Science by the following search terms: (effectiveness OR safety) AND (COVID-19 OR coronavirus OR SARS-CoV-2) AND (vaccine OR vaccination). We used EndNoteX9.0 (Thomson ResearchSoft, Stanford, USA) to manage records, screen and exclude duplicates. This study was strictly performed according to the Preferred Reporting Items for Systematic Reviews and Meta-Analyses (PRISMA).
We included observational studies that examined the effectiveness and safety of severe acute respiratory syndrome coronavirus 2 (SARS-CoV-2) vaccines among people vaccinated with SARS-CoV-2 vaccines. The following studies were excluded: (1) irrelevant to the subject of the meta-analysis, such as studies that did not use SARS-CoV-2 vaccination as the exposure; (2) insufficient data to calculate the rate for the prevention of COVID-19, the prevention of hospitalization, the prevention of admission to the ICU, the prevention of COVID-19-related death, or adverse events after vaccination; (3) duplicate studies or overlapping participants; (4) RCT studies, reviews, editorials, conference papers, case reports or animal experiments; and (5) studies that did not clarify the identification of COVID-19.
Studies were identified by two investigators (LQ and QCY) independently following the criteria above, while discrepancies reconciled by a third investigator (LJ).
Data extraction and quality assessment
The primary outcome was the effectiveness of SARS-CoV-2 vaccines. The following data were extracted independently by two investigators (LQ and QCY) from the selected studies: (1) basic information of the studies, including first author, publication year and study design; (2) characteristics of the study population, including sample sizes, age groups, setting or locations; (3) kinds of the SARS-CoV-2 vaccines; (4) outcomes for the effectiveness of SARS-CoV-2 vaccines: the number of laboratory-confirmed COVID-19, hospitalization for COVID-19, admission to the ICU for COVID-19, and COVID-19-related death; and (5) outcomes for the safety of SARS-CoV-2 vaccines: the number of adverse events after vaccination.
We evaluated the risk of bias using the Newcastle–Ottawa quality assessment scale for cohort studies and case–control studies [ 21 ]. and assess the methodological quality using the checklist recommended by Agency for Healthcare Research and Quality (AHRQ) [ 22 ]. Cohort studies and case–control studies were classified as having low (≥ 7 stars), moderate (5–6 stars), and high risk of bias (≤ 4 stars) with an overall quality score of 9 stars. For cross-sectional studies, we assigned each item of the AHRQ checklist a score of 1 (answered “yes”) or 0 (answered “no” or “unclear”), and summarized scores across items to generate an overall quality score that ranged from 0 to 11. Low, moderate, and high risk of bias were identified as having a score of 8–11, 4–7 and 0–3, respectively.
Two investigators (LQ and QCY) independently assessed study quality, with disagreements resolved by a third investigator (LJ).
Data synthesis and statistical analysis
We performed a meta-analysis to pool data from included studies and assess the effectiveness and safety of SARS-CoV-2 vaccines by clinical outcomes (rates of the prevention of COVID-19, the prevention of hospitalization, the prevention of admission to the ICU, the prevention of COVID-19-related death, and adverse events after vaccination). Random-effects or fixed-effects models were used to pool the rates and adjusted estimates across studies separately, based on the heterogeneity between estimates ( I 2 ). Fixed-effects models were used if I 2 ≤ 50%, which represented low to moderate heterogeneity and random-effects models were used if I 2 > 50%, representing substantial heterogeneity.
We conducted subgroup analyses to investigate the possible sources of heterogeneity by using vaccine kinds, vaccination status, sample size, and study population as grouping variables. We used the Q test to conduct subgroup comparisons and variables were considered significant between subgroups if the subgroup difference P value was less than 0.05. Publication bias was assessed by funnel plot and Egger’s regression test. We analyzed data using Stata version 16.0 (StataCorp, Texas, USA).
A total of 4844 records were searched from the three databases. 2484 duplicates were excluded. After reading titles and abstracts, we excluded 2264 reviews, RCT studies, duplicates and other studies meeting our exclude criteria. Among the 96 studies under full-text review, 41 studies were excluded (Fig. 1 ). Ultimately, with three grey literatures included, this final meta-analysis comprised 58 eligible studies, including 32 studies [ 14 , 15 , 17 , 18 , 19 , 20 , 23 , 24 , 25 , 26 , 27 , 28 , 29 , 30 , 31 , 32 , 33 , 34 , 35 , 36 , 37 , 38 , 39 , 40 , 41 , 42 , 43 , 44 , 45 , 46 , 47 , 48 ] for vaccine effectiveness and 26 studies [ 49 , 50 , 51 , 52 , 53 , 54 , 55 , 56 , 57 , 58 , 59 , 60 , 61 , 62 , 63 , 64 , 65 , 66 , 67 , 68 , 69 , 70 , 71 , 72 , 73 , 74 ] for vaccine safety. Characteristics of included studies are showed in Additional file 1 : Table S1, Additional file 2 : Table S2. The risk of bias of all studies we included was moderate or low.
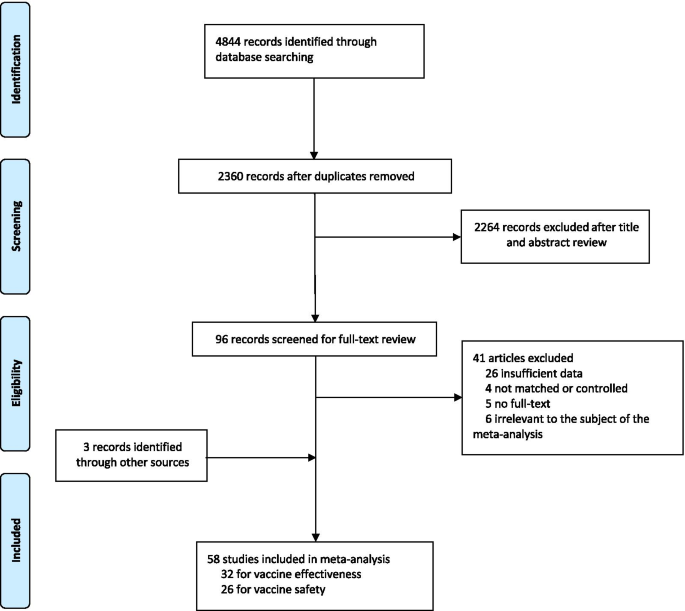
Flowchart of the study selection
Vaccine effectiveness for different clinical outcomes of COVID-19
We separately reported the vaccine effectiveness (VE) by the first and second dose of vaccines, and conducted subgroup analysis by the days after the first or second dose (< 7 days, ≥ 7 days, ≥ 14 days, and ≥ 21 days; studies with no specific days were classified as 1 dose, 2 dose or ≥ 1 dose).
For the first dose of SARS-CoV-2 vaccines, the pooled VE was 41% (95% CI : 28–54%) for the prevention of SARS-CoV-2 infection, 52% (95% CI : 31–73%) for the prevention of symptomatic COVID-19, 66% (95% CI : 50–81%) for the prevention of hospital admissions, 45% (95% CI : 42–49%) for the prevention of ICU admissions, and 53% (95% CI : 15–91%) for the prevention of COVID-19-related death (Table 1 ). The subgroup, ≥ 21 days after the first dose, was found to have the highest VE in each clinical outcome of COVID-19, regardless of ≥ 1 dose group (Table 1 ).
For the second dose of SARS-CoV-2 vaccines, the pooled VE was 85% (95% CI : 81–89%) for the prevention of SARS-CoV-2 infection, 97% (95% CI : 97–98%) for the prevention of symptomatic COVID-19, 93% (95% CI: 89–96%) for the prevention of hospital admissions, 96% (95% CI : 93–98%) for the prevention of ICU admissions, and 95% (95% CI : 92–98%) for the prevention of COVID-19-related death (Table 1 ). VE was 94% (95% CI : 78–98%) in ≥ 21 days after the second dose for the prevention of SARS-CoV-2 infection, higher than other subgroups, regardless of 2 dose group (Table 1 ). For the prevention of symptomatic COVID-19, VE was also relatively higher in 21 days after the second dose (99%, 95% CI : 94–100%). Subgroups showed no statistically significant differences in the prevention of hospital admissions, ICU admissions and COVID-19-related death (subgroup difference P values were 0.991, 0.414, and 0.851, respectively).
Vaccine effectiveness for different variants of SARS-CoV-2 in fully vaccinated people
In the fully vaccinated groups (over 14 days after the second dose), the pooled VE was 85% (95% CI: 80–91%) for the prevention of Alpha variant of SARS-CoV-2 infection, 54% (95% CI : 35–74%) for the Gamma variant, and 74% (95% CI : 62–85%) for the Delta variant. There was only one study [ 23 ] focused on the Beta variant, which showed the VE was 75% (95% CI : 71–79%) for the prevention of the Beta variant of SARS-CoV-2 infection. BNT162b2 vaccine had the highest VE in each variant group; 92% (95% CI : 90–94%) for the Alpha variant, 62% (95% CI : 2–88%) for the Gamma variant, and 84% (95% CI : 75–92%) for the Delta variant (Fig. 2 ).
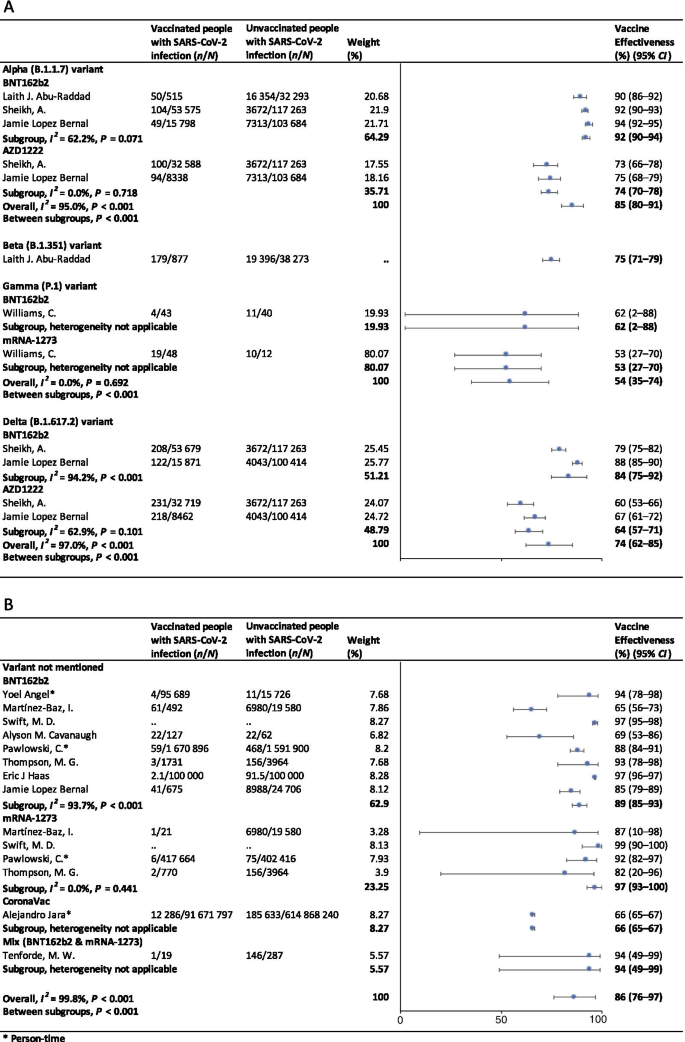
Forest plots for the vaccine effectiveness of SARS-CoV-2 vaccines in fully vaccinated populations. A Vaccine effectiveness against SARS-CoV-2 variants; B Vaccine effectiveness against SARS-CoV-2 with variants not mentioned. SARS-CoV-2 severe acute respiratory syndrome coronavirus 2, COVID-19 coronavirus disease 2019, CI confidence interval
For studies which had not mentioned the variant of SARS-CoV-2, the pooled VE was 86% (95% CI: 76–97%) for the prevention of SARS-CoV-2 infection in fully vaccinated people. mRNA-1273 vaccine had the highest pooled VE (97%, 95% CI: 93–100%, Fig. 2 ).
Safety of SARS-CoV-2 vaccines
As Table 2 showed, the incidence rate of adverse events varied widely among different studies. We conducted subgroup analysis by study population (general population, patients and healthcare workers), vaccine type (BNT162b2, mRNA-1273, CoronaVac, and et al.), and population size (< 1000, 1000–10 000, 10 000–100 000, and > 100 000). The overall pooled incidence rate was 1.5% (95% CI : 1.4–1.6%) for adverse events, 0.4 (95% CI : 0.2–0.5) per 10 000 for severe adverse events, and 0.1 (95% CI : 0.1–0.2) per 10 000 for death after vaccination. Incidence rate of adverse events was higher in healthcare workers (53.2%, 95% CI : 28.4–77.9%), AZD1222 vaccine group (79.6%, 95% CI : 60.8–98.3%), and < 1000 population size group (57.6%, 95% CI : 47.9–67.4%). Incidence rate of sever adverse events was higher in healthcare workers (127.2, 95% CI : 62.7–191.8, per 10 000), Gam-COVID-Vac vaccine group (175.7, 95% CI : 77.2–274.2, per 10 000), and 1000–10 000 population size group (336.6, 95% CI : 41.4–631.8, per 10 000). Incidence rate of death after vaccination was higher in patients (7.6, 95% CI : 0.0–32.2, per 10 000), BNT162b2 vaccine group (29.8, 95% CI : 0.0–71.2, per 10 000), and < 1000 population size group (29.8, 95% CI : 0.0–71.2, per 10 000). Subgroups of general population, vaccine type not mentioned, and > 100 000 population size had the lowest incidence rate of adverse events, severe adverse events, and death after vaccination.
Sensitivity analysis and publication bias
In the sensitivity analyses, VE for SARS-CoV-2 infections, symptomatic COVID-19 and COVID-19-related death got relatively lower when omitting over a single dose group of Maria et al.’s work [ 33 ]; when omitting ≥ 14 days after the first dose group and ≥ 14 days after the second dose group of Alejandro et al.’s work [ 14 ], VE for SARS-CoV-2 infections, hospitalization, ICU admission and COVID-19-related death got relatively higher; and VE for all clinical status of COVID-19 became lower when omitting ≥ 14 days after the second dose group of Eric et al.’s work [ 34 ]. Incidence rate of adverse events and severe adverse events got relatively higher when omitting China CDC’s data [ 74 ]. P values of Egger’s regression test for all the meta-analysis were more than 0.05, indicating that there might not be publication bias.
To our knowledge, this is a comprehensive systematic review and meta-analysis assessing the effectiveness and safety of SARS-CoV-2 vaccines based on real-world studies, reporting pooled VE for different variants of SARS-CoV-2 and incidence rate of adverse events. This meta-analysis comprised a total of 58 studies, including 32 studies for vaccine effectiveness and 26 studies for vaccine safety. We found that a single dose of SARS-CoV-2 vaccines was about 40–60% effective at preventing any clinical status of COVID-19 and that two doses were 85% or more effective. Although vaccines were not as effective against variants of SARS-CoV-2 as original virus, the vaccine effectiveness was still over 50% for fully vaccinated people. Normal adverse events were common, while the incidence of severe adverse events or even death was very low, providing reassurance to health care providers and to vaccine recipients and promote confidence in the safety of COVID-19 vaccines. Our findings strengthen and augment evidence from previous review [ 75 ], which confirmed the effectiveness of the BNT162b2 mRNA vaccine, and additionally reported the safety of SARS-CoV-2 vaccines, giving insight on the future of SARS-CoV-2 vaccine schedules.
Although most vaccines for the prevention of COVID-19 are two-dose vaccines, we found that the pooled VE of a single dose of SARS-CoV-2 vaccines was about 50%. Recent study showed that the T cell and antibody responses induced by a single dose of the BNT162b2 vaccine were comparable to those naturally infected with SARE-CoV-2 within weeks or months after infection [ 76 ]. Our findings could help to develop vaccination strategies under certain circumstances such as countries having a shortage of vaccines. In some countries, in order to administer the first dose to a larger population, the second dose was delayed for up to 12 weeks [ 77 ]. Some countries such as Canada had even decided to delay the second dose for 16 weeks [ 78 ]. However, due to a suboptimum immune response in those receiving only a single dose of a vaccine, such an approach had a chance to give rise to the emergence of variants of SARS-CoV-2 [ 79 ]. There remains a need for large clinical trials to assess the efficacy of a single-dose administration of two-dose vaccines and the risk of increasing the emergence of variants.
Two doses of SARS-CoV-2 vaccines were highly effective at preventing hospitalization, severe cases and deaths resulting from COVID-19, while the VE of different groups of days from the second vaccine dose showed no statistically significant differences. Our findings emphasized the importance of getting fully vaccinated, for the fact that most breakthrough infections were mild or asymptomatic. A recent study showed that the occurrence of breakthrough infections with SARS-CoV-2 in fully vaccinated populations was predictable with neutralizing antibody titers during the peri-infection period [ 80 ]. We also found getting fully vaccinated was at least 50% effective at preventing SARS-CoV-2 variants infections, despite reduced effectiveness compared with original virus; and BNT162b2 vaccine was found to have the highest VE in each variant group. Studies showed that the highly mutated variants were indicative of a form of rapid, multistage evolutionary jumps, which could preferentially occur in the milieu of partial immune control [ 81 , 82 ]. Therefore, immunocompromised patients should be prioritized for anti-COVID-19 immunization to mitigate persistent SARS-CoV-2 infections, during which multimutational SARS-CoV-2 variants could arise [ 83 ].
Recently, many countries, including Israel, the United States, China and the United Kingdom, have introduced a booster of COVID-19 vaccine, namely the third dose [ 84 , 85 , 86 , 87 ]. A study of Israel showed that among people vaccinated with BNT162b2 vaccine over 60 years, the risk of COVID-19 infection and severe illness in the non-booster group was 11.3 times (95% CI: 10.4–12.3) and 19.5 times (95% CI: 12.9–29.5) than the booster group, respectively [ 84 ]. Some studies have found that the third dose of Moderna, Pfizer-BioNTech, Oxford-AstraZeneca and Sinovac produced a spike in infection-blocking neutralizing antibodies when given a few months after the second dose [ 85 , 87 , 88 ]. In addition, the common adverse events associated with the third dose did not differ significantly from the symptoms of the first two doses, ranging from mild to moderate [ 85 ]. The overall incidence rate of local and systemic adverse events was 69% (57/97) and 20% (19/97) after receiving the third dose of BNT162b2 vaccine, respectively [ 88 ]. Results of a phase 3 clinical trial involving 306 people aged 18–55 years showed that adverse events after receiving a third dose of BNT162b2 vaccine (5–8 months after completion of two doses) were similar to those reported after receiving a second dose [ 85 ]. Based on V-safe, local reactions were more frequently after dose 3 (5323/6283; 84.7%) than dose 2 (5249/6283; 83.5%) among people who received 3 doses of Moderna. Systemic reactions were reported less frequently after dose 3 (4963/6283; 79.0%) than dose 2 (5105/6283; 81.3%) [ 86 ]. On August 4, WHO called for a halt to booster shots until at least the end of September to achieve an even distribution of the vaccine [ 89 ]. At this stage, the most important thing we should be thinking about is how to reach a global cover of people at risk with the first or second dose, rather than focusing on the third dose.
Based on real world studies, our results preliminarily showed that complete inoculation of COVID-19 vaccines was still effective against infection of variants, although the VE was generally diminished compared with the original virus. Particularly, the pooled VE was 54% (95% CI : 35–74%) for the Gamma variant, and 74% (95% CI : 62–85%) for the Delta variant. Since the wide spread of COVID-19, a number of variants have drawn extensive attention of international community, including Alpha variant (B.1.1.7), first identified in the United Kingdom; Beta variant (B.1.351) in South Africa; Gamma variant (P.1), initially appeared in Brazil; and the most infectious one to date, Delta variant (B.1.617.2) [ 90 ]. Israel recently reported a breakthrough infection of SARS-CoV-2, dominated by variant B.1.1.7 in a small number of fully vaccinated health care workers, raising concerns about the effectiveness of the original vaccine against those variants [ 80 ]. According to an observational cohort study in Qatar, VE of the BNT162b2 vaccine against the Alpha (B.1.1.7) and Beta (B.1.351) variants was 87% (95% CI : 81.8–90.7%) and 75.0% (95% CI : 70.5–7.9%), respectively [ 23 ]. Based on the National Immunization Management System of England, results from a recent real-world study of all the general population showed that the AZD1222 and BNT162b2 vaccines protected against symptomatic SARS-CoV-2 infection of Alpha variant with 74.5% (95% CI : 68.4–79.4%) and 93.7% (95% CI : 91.6–95.3%) [ 15 ]. In contrast, the VE against the Delta variant was 67.0% (95% CI : 61.3–71.8%) for two doses of AZD1222 vaccine and 88% (95% CI : 85.3–90.1%) for BNT162b2 vaccine [ 15 ].
In terms of adverse events after vaccination, the pooled incidence rate was very low, only 1.5% (95% CI : 1.4–1.6%). However, the prevalence of adverse events reported in large population (population size > 100 000) was much lower than that in small to medium population size. On the one hand, the vaccination population in the small to medium scale studies we included were mostly composed by health care workers, patients with specific diseases or the elderly. And these people are more concerned about their health and more sensitive to changes of themselves. But it remains to be proved whether patients or the elderly are more likely to have adverse events than the general. Mainstream vaccines currently on the market have maintained robust safety in specific populations such as cancer patients, organ transplant recipients, patients with rheumatic and musculoskeletal diseases, pregnant women and the elderly [ 54 , 91 , 92 , 93 , 94 ]. A prospective study by Tal Goshen-lag suggests that the safety of BNT162b2 vaccine in cancer patients is consistent with those previous reports [ 91 ]. In addition, the incidence rate of adverse events reported in the heart–lung transplant population is even lower than that in general population [ 95 ]. On the other hand, large scale studies at the national level are mostly based on national electronic health records or adverse event reporting systems, and it is likely that most mild or moderate symptoms are actually not reported.
Compared with the usual local adverse events (such as pain at the injection site, redness at the injection site, etc.) and normal systemic reactions (such as fatigue, myalgia, etc.), serious and life-threatening adverse events were rare due to our results. A meta-analysis based on RCTs only showed three cases of anaphylactic shock among 58 889 COVID-19 vaccine recipients and one in the placebo group [ 11 ]. The exact mechanisms underlying most of the adverse events are still unclear, accordingly we cannot establish a causal relation between severe adverse events and vaccination directly based on observational studies. In general, varying degrees of adverse events occur after different types of COVID-19 vaccination. Nevertheless, the benefits far outweigh the risks.
Our results showed the effectiveness and safety of different types of vaccines varied greatly. Regardless of SARS-CoV-2 variants, vaccine effectiveness varied from 66% (CoronaVac [ 14 ]) to 97% (mRNA-1273 [ 18 , 20 , 45 , 46 ]). The incidence rate of adverse events varied widely among different types of vaccines, which, however, could be explained by the sample size and population group of participants. BNT162b2, AZD1222, mRNA-1273 and CoronaVac were all found to have high vaccine efficacy and acceptable adverse-event profile in recent published studies [ 96 , 97 , 98 , 99 ]. A meta-analysis, focusing on the potential vaccine candidate which have reached to the phase 3 of clinical development, also found that although many of the vaccines caused more adverse events than the controls, most were mild, transient and manageable [ 100 ]. However, severe adverse events did occur, and there remains the need to implement a unified global surveillance system to monitor the adverse events of COVID-19 vaccines around the world [ 101 ]. A recent study employed a knowledge-based or rational strategy to perform a prioritization matrix of approved COVID-19 vaccines, and led to a scale with JANSSEN (Ad26.COV2.S) in the first place, and AZD1222, BNT162b2, and Sputnik V in second place, followed by BBIBP-CorV, CoronaVac and mRNA-1273 in third place [ 101 ]. Moreover, when deciding the priority of vaccines, the socioeconomic characteristics of each country should also be considered.
Our meta-analysis still has several limitations. First, we may include limited basic data on specific populations, as vaccination is slowly being promoted in populations under the age of 18 or over 60. Second, due to the limitation of the original real-world study, we did not conduct subgroup analysis based on more population characteristics, such as age. When analyzing the efficacy and safety of COVID-19 vaccine, we may have neglected the discussion on the heterogeneity from these sources. Third, most of the original studies only collected adverse events within 7 days after vaccination, which may limit the duration of follow-up for safety analysis.
Based on the real-world studies, SARS-CoV-2 vaccines have reassuring safety and could effectively reduce the death, severe cases, symptomatic cases, and infections resulting from SARS-CoV-2 across the world. In the context of global pandemic and the continuous emergence of SARS-CoV-2 variants, accelerating vaccination and improving vaccination coverage is still the most important and urgent matter, and it is also the final means to end the pandemic.
Availability of data and materials
All data generated or analyzed during this study are included in this published article and its additional information files.
Abbreviations
Coronavirus disease 2019
Severe Acute Respiratory Syndrome Coronavirus 2
Vaccine effectiveness
Confidence intervals
Intensive care unit
Random clinical trials
Preferred reporting items for systematic reviews and meta-analyses
COVID-19 Dashboard by the Center for Systems Science and Engineering (CSSE) at Johns Hopkins University (JHU). 2021. https://coronavirus.jhu.edu/map.html . Accessed 20 Aug 2021.
Barranco R, Rocca G, Molinelli A, Ventura F. Controversies and challenges of mass vaccination against SARS-CoV-2 in Italy: medico-legal perspectives and considerations. Healthcare (Basel). 2021. https://doi.org/10.3390/healthcare9091163 .
Article Google Scholar
COVID-19 vaccine tracker. 2021. https://vac-lshtm.shinyapps.io/ncov_vaccine_landscape/ . Accessed 20 Aug 2021.
Coronavirus (COVID-19) Vaccinations. 2021. https://ourworldindata.org/covid-vaccinations . Accessed 20 Aug 2021.
Kirby T. New variant of SARS-CoV-2 in UK causes surge of COVID-19. Lancet Respir Med. 2021;9(2):e20–1. https://doi.org/10.1016/s2213-2600(21)00005-9 .
Article CAS PubMed PubMed Central Google Scholar
Callaway E. Fast-spreading COVID variant can elude immune responses. Nature. 2021;589(7843):500–1. https://doi.org/10.1038/d41586-021-00121-z .
Article CAS PubMed Google Scholar
Reardon S. How the Delta variant achieves its ultrafast spread. Nature. 2021. https://doi.org/10.1038/d41586-021-01986-w .
Article PubMed Google Scholar
Li R, Liu J, Zhang H. The challenge of emerging SARS-CoV-2 mutants to vaccine development. J Genet Genomics. 2021;48(2):102–6. https://doi.org/10.1016/j.jgg.2021.03.001 .
Article PubMed PubMed Central Google Scholar
Chen M, Yuan Y, Zhou Y, Deng Z, Zhao J, Feng F, Zou H, Sun C. Safety of SARS-CoV-2 vaccines: a systematic review and meta-analysis of randomized controlled trials. Infect Dis Poverty. 2021;10(1):94. https://doi.org/10.1186/s40249-021-00878-5 .
Ling Y, Zhong J, Luo J. Safety and effectiveness of SARS-CoV-2 vaccines: a systematic review and meta-analysis. J Med Virol. 2021. https://doi.org/10.1002/jmv.27203 .
Pormohammad A, Zarei M, Ghorbani S, Mohammadi M, Razizadeh MH, Turner DL, Turner RJ. Efficacy and safety of COVID-19 vaccines: a systematic review and meta-analysis of randomized clinical trials. Vaccines (Basel). 2021. https://doi.org/10.3390/vaccines9050467 .
Sathian B, Asim M, Banerjee I, Roy B, Pizarro AB, Mancha MA, van Teijlingen ER, Kord-Varkaneh H, Mekkodathil AA, Subramanya SH, et al. Development and implementation of a potential coronavirus disease 2019 (COVID-19) vaccine: a systematic review and meta-analysis of vaccine clinical trials. Nepal J Epidemiol. 2021;11(1):959–82. https://doi.org/10.3126/nje.v11i1.36163 .
Yuan P, Ai P, Liu Y, Ai Z, Wang Y, Cao W, Xia X, Zheng JC. Safety, tolerability, and immunogenicity of COVID-19 vaccines: a systematic review and meta-analysis. medRxiv. 2020. https://doi.org/10.1101/2020.11.03.20224998 .
Jara A, Undurraga EA, González C, Paredes F, Fontecilla T, Jara G, Pizarro A, Acevedo J, Leo K, Leon F, et al. Effectiveness of an inactivated SARS-CoV-2 vaccine in Chile. N Engl J Med. 2021. https://doi.org/10.1056/NEJMoa2107715 .
Lopez Bernal J, Andrews N, Gower C, Gallagher E, Simmons R, Thelwall S, Stowe J, Tessier E, Groves N, Dabrera G, et al. Effectiveness of COVID-19 vaccines against the B.1.617.2 (Delta) variant. N Engl J Med. 2021. https://doi.org/10.1056/NEJMoa2108891 .
Israel says Pfizer Covid vaccine is just 39% effective as delta spreads, but still prevents severe illness. 2021. https://www.cnbc.com/2021/07/23/delta-variant-pfizer-covid-vaccine-39percent-effective-in-israel-prevents-severe-illness.html . Accessed 20 Aug 2021.
Zacay G, Shasha D, Bareket R, Kadim I, Hershkowitz Sikron F, Tsamir J, Mossinson D, Heymann AD. BNT162b2 vaccine effectiveness in preventing asymptomatic infection with SARS-CoV-2 virus: a nationwide historical cohort study. Open Forum Infect Dis. 2021;8(6): ofab262. https://doi.org/10.1093/ofid/ofab262 .
Martínez-Baz I, Miqueleiz A, Casado I, Navascués A, Trobajo-Sanmartín C, Burgui C, Guevara M, Ezpeleta C, Castilla J. Effectiveness of COVID-19 vaccines in preventing SARS-CoV-2 infection and hospitalisation, Navarre, Spain, January to April 2021. Eurosurveillance. 2021. https://doi.org/10.2807/1560-7917.Es.2021.26.21.2100438 .
Tenforde MW, Olson SM, Self WH, Talbot HK, Lindsell CJ, Steingrub JS, Shapiro NI, Ginde AA, Douin DJ, Prekker ME, et al. Effectiveness of Pfizer-BioNTech and moderna vaccines against COVID-19 among hospitalized adults aged ≥65 years—United States, January–March 2021. MMWR Morb Mortal Wkly Rep. 2021;70(18):674–9. https://doi.org/10.15585/mmwr.mm7018e1 .
Pawlowski C, Lenehan P, Puranik A, Agarwal V, Venkatakrishnan AJ, Niesen MJM, O’Horo JC, Virk A, Swift MD, Badley AD, et al. FDA-authorized mRNA COVID-19 vaccines are effective per real-world evidence synthesized across a multi-state health system. Med (N Y). 2021. https://doi.org/10.1016/j.medj.2021.06.007 .
Wells G, Shea B, O'Connell D, Peterson J, Welch V, Losos M, Tugwell P. The Newcastle-Ottawa Scale (NOS) for assessing the quality of nonrandomised studies in meta-analyses. http://www.ohri.ca/programs/clinical_epidemiology/oxford.asp . Accessed 20 Aug 2021.
Rostom A, Dubé C, Cranney A, et al. Celiac Disease. Rockville (MD): Agency for Healthcare Research and Quality (US); 2004 Sep. (Evidence Reports/Technology Assessments, No. 104.) Appendix D. Quality Assessment Forms. Available from: https://www.ncbi.nlm.nih.gov/books/NBK35156/ . Accessed 20 Aug 2021
Abu-Raddad LJ, Chemaitelly H, Butt AA. Effectiveness of the BNT162b2 COVID-19 vaccine against the B.1.1.7 and B.1.351 Variants. N Engl J Med. 2021;385(2):187–9. https://doi.org/10.1056/NEJMc2104974 .
Angel Y, Spitzer A, Henig O, Saiag E, Sprecher E, Padova H, Ben-Ami R. Association between vaccination with BNT162b2 and incidence of symptomatic and asymptomatic SARS-CoV-2 infections among health care workers. JAMA. 2021;325(24):2457–65. https://doi.org/10.1001/jama.2021.7152 .
Azamgarhi T, Hodgkinson M, Shah A, Skinner JA, Hauptmannova I, Briggs TWR, Warren S. BNT162b2 vaccine uptake and effectiveness in UK healthcare workers—a single centre cohort study. Nat Commun. 2021;12(1):3698. https://doi.org/10.1038/s41467-021-23927-x .
Bianchi FP, Germinario CA, Migliore G, Vimercati L, Martinelli A, Lobifaro A, Tafuri S, Stefanizzi P. BNT162b2 mRNA COVID-19 vaccine effectiveness in the prevention of SARS-CoV-2 infection: a preliminary report. J Infect Dis. 2021. https://doi.org/10.1093/infdis/jiab262 .
Britton A, Jacobs Slifka KM, Edens C, Nanduri SA, Bart SM, Shang N, Harizaj A, Armstrong J, Xu K, Ehrlich HY, et al. Effectiveness of the Pfizer-BioNTech COVID-19 vaccine among residents of two skilled nursing facilities experiencing COVID-19 outbreaks—Connecticut, December 2020–February 2021. MMWR Morb Mortal Wkly Rep. 2021;70(11):396–401. https://doi.org/10.15585/mmwr.mm7011e3 .
Cavanaugh AM, Fortier S, Lewis P, Arora V, Johnson M, George K, Tobias J, Lunn S, Miller T, Thoroughman D, et al. COVID-19 outbreak associated with a SARS-CoV-2 R1 lineage variant in a skilled nursing facility after vaccination program—Kentucky, March 2021. MMWR Morb Mortal Wkly Rep. 2021;70(17):639–43. https://doi.org/10.15585/mmwr.mm7017e2 .
Chemaitelly H, Yassine HM, Benslimane FM, Al Khatib HA, Tang P, Hasan MR, Malek JA, Coyle P, Ayoub HH, Al Kanaani Z, et al. mRNA-1273 COVID-19 vaccine effectiveness against the B.1.1.7 and B.1.351 variants and severe COVID-19 disease in Qatar. Nat Med. 2021. https://doi.org/10.1038/s41591-021-01446-y .
Chodick G, Tene L, Patalon T, Gazit S, Ben Tov A, Cohen D, Muhsen K. Assessment of effectiveness of 1 dose of BNT162b2 vaccine for SARS-CoV-2 infection 13 to 24 days after immunization. JAMA Netw Open. 2021;4(6): e2115985. https://doi.org/10.1001/jamanetworkopen.2021.15985 .
Chodick G, Tene L, Rotem RS, Patalon T, Gazit S, Ben-Tov A, Weil C, Goldshtein I, Twig G, Cohen D, et al. The effectiveness of the TWO-DOSE BNT162b2 vaccine: analysis of real-world data. Clin Infect Dis. 2021. https://doi.org/10.1093/cid/ciab438 .
Dagan N, Barda N, Kepten E, Miron O, Perchik S, Katz MA, Hernán MA, Lipsitch M, Reis B, Balicer RD. BNT162b2 mRNA COVID-19 vaccine in a nationwide mass vaccination setting. N Engl J Med. 2021;384(15):1412–23. https://doi.org/10.1056/NEJMoa2101765 .
Flacco ME, Soldato G, Acuti Martellucci C, Carota R, Di Luzio R, Caponetti A, Manzoli L. Interim estimates of COVID-19 vaccine effectiveness in a mass vaccination setting: data from an Italian Province. VacCInes (Basel). 2021. https://doi.org/10.3390/vaccines9060628 .
Haas EJ, Angulo FJ, McLaughlin JM, Anis E, Singer SR, Khan F, Brooks N, Smaja M, Mircus G, Pan K, et al. Impact and effectiveness of mRNA BNT162b2 vaccine against SARS-CoV-2 infections and COVID-19 cases, hospitalisations, and deaths following a nationwide vaccination campaign in Israel: an observational study using national surveillance data. Lancet. 2021;397(10287):1819–29. https://doi.org/10.1016/s0140-6736(21)00947-8 .
Hall VJ, Foulkes S, Saei A, Andrews N, Oguti B, Charlett A, Wellington E, Stowe J, Gillson N, Atti A, et al. COVID-19 vaccine coverage in health-care workers in England and effectiveness of BNT162b2 mRNA vaccine against infection (SIREN): a prospective, multicentre, cohort study. Lancet. 2021;397(10286):1725–35. https://doi.org/10.1016/s0140-6736(21)00790-x .
Hyams C, Marlow R, Maseko Z, King J, Ward L, Fox K, Heath R, Tuner A, Friedrich Z, Morrison L, et al. Effectiveness of BNT162b2 and ChAdOx1 nCoV-19 COVID-19 vaccination at preventing hospitalisations in people aged at least 80 years: a test-negative, case-control study. Lancet Infect Dis. 2021. https://doi.org/10.1016/s1473-3099(21)00330-3 .
Khan N, Mahmud N. Effectiveness of SARS-CoV-2 vaccination in a veterans affairs cohort of patients with inflammatory bowel disease with diverse exposure to immunosuppressive medications. Gastroenterology. 2021. https://doi.org/10.1053/j.gastro.2021.05.044 .
Knobel P, Serra C, Grau S, Ibañez R, Diaz P, Ferrández O, Villar R, Lopez AF, Pujolar N, Horcajada JP, et al. COVID-19 mRNA vaccine effectiveness in asymptomatic healthcare workers. Infect Control Hosp Epidemiol. 2021. https://doi.org/10.1017/ice.2021.287 .
Lopez Bernal J, Andrews N, Gower C, Robertson C, Stowe J, Tessier E, Simmons R, Cottrell S, Roberts R, O’Doherty M, et al. Effectiveness of the Pfizer-BioNTech and Oxford-AstraZeneca vaccines on covid-19 related symptoms, hospital admissions, and mortality in older adults in England: test negative case-control study. BMJ. 2021;373: n1088. https://doi.org/10.1136/bmj.n1088 .
Mazagatos C, Monge S, Olmedo C, Vega L, Gallego P, Martín-Merino E, Sierra MJ, Limia A, Larrauri A. Effectiveness of mRNA COVID-19 vaccines in preventing SARS-CoV-2 infections and COVID-19 hospitalisations and deaths in elderly long-term care facility residents, Spain, weeks 53, 2020 to 13 2021. Eurosurveillance. 2021. https://doi.org/10.2807/1560-7917.Es.2021.26.24.2100452 .
Pilishvili T, Fleming-Dutra KE, Farrar JL, Gierke R, Mohr NM, Talan DA, Krishnadasan A, Harland KK, Smithline HA, Hou PC, et al. Interim estimates of vaccine effectiveness of Pfizer-BioNTech and Moderna COVID-19 vaccines among health care personnel—33 US Sites, January–March 2021. MMWR Morb Mortal Wkly Rep. 2021;70(20):753–8. https://doi.org/10.15585/mmwr.mm7020e2 .
Sheikh A, McMenamin J, Taylor B, Robertson C. SARS-CoV-2 Delta VOC in Scotland: demographics, risk of hospital admission, and vaccine effectiveness. Lancet. 2021;397(10293):2461–2. https://doi.org/10.1016/s0140-6736(21)01358-1 .
Shrotri M, Krutikov M, Palmer T, Giddings R, Azmi B, Subbarao S, Fuller C, Irwin-Singer A, Davies D, Tut G, et al. Vaccine effectiveness of the first dose of ChAdOx1 nCoV-19 and BNT162b2 against SARS-CoV-2 infection in residents of long-term care facilities in England (VIVALDI): a prospective cohort study. Lancet Infect Dis. 2021. https://doi.org/10.1016/s1473-3099(21)00289-9 .
Skowronski DM, Setayeshgar S, Zou M, Prystajecky N, Tyson JR, Galanis E, Naus M, Patrick DM, Sbihi H, El Adam S, et al. Single-dose mRNA vaccine effectiveness against SARS-CoV-2, including Alpha and Gamma variants: a test-negative design in adults 70 years and older in British Columbia,Canada. Clin Infect Dis. 2021. https://doi.org/10.1093/cid/ciab616 .
Swift MD, Breeher LE, Tande AJ, Tommaso CP, Hainy CM, Chu H, Murad MH, Berbari EF, Virk A. Effectiveness of mRNA COVID-19 vaccines against SARS-CoV-2 infection in a cohort of healthcare personnel. Clin Infect Dis. 2021. https://doi.org/10.1093/cid/ciab361 .
Thompson MG, Burgess JL, Naleway AL, Tyner H, Yoon SK, Meece J, Olsho LEW, Caban-Martinez AJ, Fowlkes AL, Lutrick K, et al. Prevention and attenuation of COVID-19 with the BNT162b2 and mRNA-1273 Vaccines. N Engl J Med. 2021. https://doi.org/10.1056/NEJMoa2107058 .
Vasileiou E, Simpson CR, Shi T, Kerr S, Agrawal U, Akbari A, Bedston S, Beggs J, Bradley D, Chuter A, et al. Interim findings from first-dose mass COVID-19 vaccination roll-out and COVID-19 hospital admissions in Scotland: a national prospective cohort study. Lancet. 2021;397(10285):1646–57. https://doi.org/10.1016/s0140-6736(21)00677-2 .
Williams C, Al-Bargash D, Macalintal C, Stuart R, Seth A, Latham J, Gitterman L, Fedsin S, Godoy M, Kozak R, et al. COVID-19 outbreak associated with a SARS-CoV-2 P.1 lineage in a long-term care home after implementation of a vaccination program—Ontario, April–May 2021. Clin Infect Dis. 2021. https://doi.org/10.1093/cid/ciab617 .
Alhazmi A, Alamer E, Daws D, Hakami M, Darraj M, Abdelwahab S, Maghfuri A, Algaissi A. Evaluation of side effects associated with COVID-19 vaccines in Saudi Arabia. Vaccines (Basel). 2021. https://doi.org/10.3390/vaccines9060674 .
Andrzejczak-Grządko S, Czudy Z, Donderska M. Side effects after COVID-19 vaccinations among residents of Poland. Eur Rev Med Pharmacol Sci. 2021;25(12):4418–21. https://doi.org/10.26355/eurrev_202106_26153 .
Baldolli A, Michon J, Appia F, Galimard C, Verdon R, Parienti JJ. Tolerance of BNT162b2 mRNA COVI-19 vaccine in patients with a medical history of COVID-19 disease: a case control study. Vaccine. 2021;39(32):4410–3. https://doi.org/10.1016/j.vaccine.2021.06.054 .
Cherian S, Paul A, Ahmed S, Alias B, Manoj M, Santhosh AK, Varghese DR, Krishnan N, Shenoy P. Safety of the ChAdOx1 nCoV-19 and the BBV152 vaccines in 724 patients with rheumatic diseases: a post-vaccination cross-sectional survey. Rheumatol Int. 2021;41(8):1441–5. https://doi.org/10.1007/s00296-021-04917-0 .
Chevallier P, Coste-Burel M, Le Bourgeois A, Peterlin P, Garnier A, Béné MC, Imbert BM, Drumel T, Le Gouill S, Moreau P, et al. Safety and immunogenicity of a first dose of SARS-CoV-2 mRNA vaccine in allogeneic hematopoietic stem-cells recipients. EJHaem. 2021. https://doi.org/10.1002/jha2.242 .
Connolly CM, Ruddy JA, Boyarsky BJ, Avery RK, Werbel WA, Segev DL, Garonzik-Wang J, Paik JJ. Safety of the first dose of mRNA SARS-CoV-2 vaccines in patients with rheumatic and musculoskeletal diseases. Ann Rheum Dis. 2021. https://doi.org/10.1136/annrheumdis-2021-220231 .
Furer V, Eviatar T, Zisman D, Peleg H, Paran D, Levartovsky D, Zisapel M, Elalouf O, Kaufman I, Meidan R, et al. Immunogenicity and safety of the BNT162b2 mRNA COVID-19 vaccine in adult patients with autoimmune inflammatory rheumatic diseases and in the general population: a multicentre study. Ann Rheum Dis. 2021. https://doi.org/10.1136/annrheumdis-2021-220647 .
Gee J, Marquez P, Su J, Calvert GM, Liu R, Myers T, Nair N, Martin S, Clark T, Markowitz L, et al. First month of COVID-19 vaccine safety monitoring—United States, December 14, 2020–January 13, 2021. MMWR Morb Mortal Wkly Rep. 2021;70(8):283–8. https://doi.org/10.15585/mmwr.mm7008e3 .
Hashimoto T, Ozaki A, Bhandari D, Sawano T, Sah R, Tanimoto T. High anaphylaxis rates following vaccination with the Pfizer BNT162b2 mRNA vaccine against COVID-19 in Japanese health care workers; a secondary analysis of initial post-approval safety data. J Travel Med. 2021. https://doi.org/10.1093/jtm/taab090 .
Lv G, Yuan J, Xiong X, Li M. Mortality rate and characteristics of deaths following COVID-19 vaccination. Front Med (Lausanne). 2021;8: 670370. https://doi.org/10.3389/fmed.2021.670370 .
McMurry R, Lenehan P, Awasthi S, Silvert E, Puranik A, Pawlowski C, Venkatakrishnan AJ, Anand P, Agarwal V, O’Horo JC, et al. Real-time analysis of a mass vaccination effort confirms the safety of FDA-authorized mRNA COVID-19 vaccines. Med (N Y). 2021. https://doi.org/10.1016/j.medj.2021.06.006 .
Monin L, Laing AG, Muñoz-Ruiz M, McKenzie DR, Del Molino Del Barrio I, Alaguthurai T, Domingo-Vila C, Hayday TS, Graham C, Seow J, et al. Safety and immunogenicity of one versus two doses of the COVID-19 vaccine BNT162b2 for patients with cancer: interim analysis of a prospective observational study. Lancet Oncol. 2021;22(6):765–78. https://doi.org/10.1016/s1470-2045(21)00213-8 .
Pagotto V, Ferloni A, Mercedes Soriano M, Díaz M, Braguinsky Golde N, González MI, Asprea V, Staneloni MI, Zingoni P, Vidal G, et al. Active monitoring of early safety of Sputnik V vaccine in Buenos Aires, Argentina. MediCIna (B Aires). 2021;81(3):408–14.
Google Scholar
Peled Y, Ram E, Lavee J, Sternik L, Segev A, Wieder-Finesod A, Mandelboim M, Indenbaum V, Levy I, Raanani E, et al. BNT162b2 vaccination in heart transplant recipients: Clinical experience and antibody response. J Heart Lung Transplant. 2021. https://doi.org/10.1016/j.healun.2021.04.003 .
Quiroga B, Sánchez-Álvarez E, Goicoechea M, de Sequera P. COVID-19 vaccination among Spanish nephrologists: acceptance and side effects. J Healthc Qual Res. 2021. https://doi.org/10.1016/j.jhqr.2021.05.002 .
Ram R, Hagin D, Kikozashvilli N, Freund T, Amit O, Bar-On Y, Beyar-Katz O, Shefer G, Moshiashvili MM, Karni C, et al. Safety and immunogenicity of the BNT162b2 mRNA COVID-19 vaccine in patients after allogeneic HCT or CD19-based CART therapy—a single center prospective cohort study. Transplant Cell Ther. 2021. https://doi.org/10.1016/j.jtct.2021.06.024 .
Revon-Riviere G, Ninove L, Min V, Rome A, Coze C, Verschuur A, de Lamballerie X, André N. The BNT162b2 mRNA COVID-19 vaccine in adolescents and young adults with cancer: a monocentric experience. Eur J Cancer. 2021;154:30–4. https://doi.org/10.1016/j.ejca.2021.06.002 .
Riad A, Pokorná A, Mekhemar M, Conrad J, Klugarová J, Koščík M, Klugar M, Attia S. Safety of ChAdOx1 nCoV-19 vaccine: independent evidence from two EU states. Vaccines (Basel). 2021. https://doi.org/10.3390/vaccines9060673 .
Riad A, Sağıroğlu D, Üstün B, Pokorná A, Klugarová J, Attia S, Klugar M. Prevalence and risk factors of CoronaVac Side effects: an independent cross-sectional study among healthcare workers in Turkey. J Clin Med. 2021. https://doi.org/10.3390/jcm10122629 .
Rosman Y, Lavi N, Meir-Shafrir K, Lachover-Roth I, Cohen-Engler A, Mekori YA, Confino-Cohen R. Safety of BNT162b2 mRNA COVID-19 vaccine in patients with mast cell disorders. J Allergy Clin Immunol Pract. 2021. https://doi.org/10.1016/j.jaip.2021.06.032 .
Signorelli C, Odone A, Gianfredi V, Capraro M, Kacerik E, Chiecca G, Scardoni A, Minerva M, Mantecca R, Musarò P, et al. Application of the “immunization islands” model to improve quality, efficiency and safety of a COVID-19 mass vaccination site. Ann Ig. 2021;33(5):499–512. https://doi.org/10.7416/ai.2021.2456 .
Vallée A, Chan-Hew-Wai A, Bonan B, Lesprit P, Parquin F, Catherinot É, Choucair J, Billard D, Amiel-Taieb C, Camps È, et al. Oxford-AstraZeneca COVID-19 vaccine: need of a reasoned and effective vaccine campaign. Public Health. 2021;196:135–7. https://doi.org/10.1016/j.puhe.2021.05.030 .
Wang J, Hou Z, Liu J, Gu Y, Wu Y, Chen Z, Ji J, Diao S, Qiu Y, Zou S, et al. Safety and immunogenicity of COVID-19 vaccination in patients with non-alcoholic fatty liver disease (CHESS2101): a multicenter study. J Hepatol. 2021. https://doi.org/10.1016/j.jhep.2021.04.026 .
Zhang MX, Zhang TT, Shi GF, Cheng FM, Zheng YM, Tung TH, Chen HX. Safety of an inactivated SARS-CoV-2 vaccine among healthcare workers in China. Expert Rev Vaccines. 2021. https://doi.org/10.1080/14760584.2021.1925112 .
Shay DK, Gee J, Su JR, Myers TR, Marquez P, Liu R, Zhang B, Licata C, Clark TA, Shimabukuro TT. Safety monitoring of the Janssen (Johnson & Johnson) COVID-19 Vaccine—United States, March–April 2021. MMWR Morb Mortal Wkly Rep. 2021;70(18):680–4. https://doi.org/10.15585/mmwr.mm7018e2 .
Prevention CCfDCa. Information analysis of COVID-19 vaccine adverse reaction monitoring in China. 2021-5-28. http://www.chinacdc.cn/jkzt/ymyjz/ymyjjz_6758/202105/t20210528_230908.html . Accessed 20 Aug 2021.
Kow CS, Hasan SS. Real-world effectiveness of BNT162b2 mRNA vaccine: a meta-analysis of large observational studies. Inflammopharmacology. 2021;29(4):1075–90. https://doi.org/10.1007/s10787-021-00839-2 .
Angyal A, Longet S, Moore S, Payne RP, Harding A et al. T-Cell and Antibody Responses to First BNT162b2 Vaccine Dose in Previously SARS-CoV-2-Infected and Infection-Naive UK Healthcare Workers: A Multicentre, Prospective, Observational Cohort Study. Available at SSRN: https://ssrn.com/abstract=3820576 or https://doi.org/10.2139/ssrn.3820576 . Accessed 20 Aug 2021.
Pimenta D, Yates C, Pagel C, Gurdasani D. Delaying the second dose of covid-19 vaccines. BMJ. 2021;372: n710. https://doi.org/10.1136/bmj.n710 .
Tauh T, Mozel M, Meyler P, Lee SM. An updated look at the 16-week window between doses of vaccines in BC for COVID-19. BC Med J. 2021;63(3):102–3.
Kadire SR, Wachter RM, Lurie N. Delayed second dose versus standard regimen for COVID-19 vaccination. N Engl J Med. 2021;384(9): e28. https://doi.org/10.1056/NEJMclde2101987 .
Bergwerk M, Gonen T, Lustig Y, Amit S, Lipsitch M, Cohen C, Mandelboim M, Gal Levin E, Rubin C, Indenbaum V, et al. COVID-19 breakthrough infections in vaccinated health care workers. N Engl J Med. 2021. https://doi.org/10.1056/NEJMoa2109072 .
Truong TT, Ryutov A, Pandey U, Yee R, Goldberg L, Bhojwani D, Aguayo-Hiraldo P, Pinsky BA, Pekosz A, Shen L, et al. Persistent SARS-CoV-2 infection and increasing viral variants in children and young adults with impaired humoral immunity. medRxiv. 2021. https://doi.org/10.1101/2021.02.27.21252099 .
Choi B, Choudhary MC, Regan J, Sparks JA, Padera RF, Qiu X, Solomon IH, Kuo HH, Boucau J, Bowman K, et al. Persistence and evolution of SARS-CoV-2 in an Immunocompromised Host. N Engl J Med. 2020;383(23):2291–3. https://doi.org/10.1056/NEJMc2031364 .
Corey L, Beyrer C, Cohen MS, Michael NL, Bedford T, Rolland M. SARS-CoV-2 variants in patients with immunosuppression. N Engl J Med. 2021;385(6):562–6. https://doi.org/10.1056/NEJMsb2104756 .
Bar-On YM, Goldberg Y, Mandel M, Bodenheimer O, Freedman L, Kalkstein N, Mizrahi B, Alroy-Preis S, Ash N, Milo R, et al. Protection of BNT162b2 vaccine booster against Covid-19 in Israel. N Engl J Med. 2021;385(15):1393–400. https://doi.org/10.1056/NEJMoa2114255 .
Hause AM, Baggs J, Gee J, Marquez P, Myers TR, Shimabukuro TT, Shay DK. Safety monitoring of an additional dose of COVID-19 vaccine—United States, August 12–September 19, 2021. MMWR Morb Mortal Wkly Rep. 2021;70(39):1379–84. https://doi.org/10.15585/mmwr.mm7039e4 .
Furlow B. Immunocompromised patients in the USA and UK should receive third dose of COVID-19 vaccine. Lancet Rheumatol. 2021. https://doi.org/10.1016/s2665-9913(21)00313-1 .
Flaxman A, Marchevsky NG, Jenkin D, Aboagye J, Aley PK, Angus B, Belij-Rammerstorfer S, Bibi S, Bittaye M, Cappuccini F, et al. Reactogenicity and immunogenicity after a late second dose or a third dose of ChAdOx1 nCoV-19 in the UK: a substudy of two randomised controlled trials (COV001 and COV002). Lancet. 2021;398(10304):981–90. https://doi.org/10.1016/s0140-6736(21)01699-8 .
Peled Y, Ram E, Lavee J, Segev A, Matezki S, Wieder-Finesod A, Halperin R, Mandelboim M, Indenbaum V, Levy I, et al. Third dose of the BNT162b2 vaccine in heart transplant recipients: immunogenicity and clinical experience. J Heart Lung Transplant. 2021. https://doi.org/10.1016/j.healun.2021.08.010 .
WHO. WHO press conference on coronavirus disease (COVID-19)—4 August 2021. 2021. https://www.who.int/multi-media/details/who-press-conference-on-coronavirus-disease-(covid-19)---4-august-2021 . Accessed 20 Aug 2021.
Cascella M, Rajnik M, Aleem A, Dulebohn SC, Di Napoli R. Features, evaluation, and treatment of coronavirus (COVID-19). In: StatPearls. edn. Treasure Island (FL): StatPearls Publishing Copyright © 2021, StatPearls Publishing LLC.; 2021.
Goshen-Lago T, Waldhorn I, Holland R, Szwarcwort-Cohen M, Reiner-Benaim A, Shachor-Meyouhas Y, Hussein K, Fahoum L, Baruch M, Peer A, et al. Serologic status and toxic effects of the SARS-CoV-2 BNT162b2 vaccine in patients undergoing treatment for cancer. JAMA Oncol. 2021. https://doi.org/10.1001/jamaoncol.2021.2675 .
Ou MT, Boyarsky BJ, Motter JD, Greenberg RS, Teles AT, Ruddy JA, Krach MR, Jain VS, Werbel WA, Avery RK, et al. Safety and reactogenicity of 2 doses of SARS-CoV-2 vaccination in solid organ transplant recipients. Transplantation. 2021. https://doi.org/10.1097/tp.0000000000003780 .
Bookstein Peretz S, Regev N, Novick L, Nachshol M, Goffer E, Ben-David A, Asraf K, Doolman R, Sapir E, Regev Yochay G, et al. Short-term outcome of pregnant women vaccinated by BNT162b2 mRNA COVID-19 vaccine. Ultrasound Obstet Gynecol. 2021. https://doi.org/10.1002/uog.23729 .
Shimabukuro TT, Kim SY, Myers TR, Moro PL, Oduyebo T, Panagiotakopoulos L, Marquez PL, Olson CK, Liu R, Chang KT, et al. Preliminary findings of mRNA COVID-19 vaccine safety in pregnant persons. N Engl J Med. 2021;384(24):2273–82. https://doi.org/10.1056/NEJMoa2104983 .
Peled Y, Ram E, Lavee J, Sternik L, Segev A, Wieder-Finesod A, Mandelboim M, Indenbaum V, Levy I, Raanani E, et al. BNT162b2 vaccination in heart transplant recipients: clinical experience and antibody response. J Heart Lung Transplant. 2021;40(8):759–62. https://doi.org/10.1016/j.healun.2021.04.003 .
Thomas SJ, Moreira ED Jr, Kitchin N, Absalon J, Gurtman A, Lockhart S, Perez JL, Pérez Marc G, Polack FP, Zerbini C, et al. Safety and efficacy of the BNT162b2 mRNA COVID-19 vaccine through 6 months. N Engl J Med. 2021. https://doi.org/10.1056/NEJMoa2110345 .
Falsey AR, Sobieszczyk ME, Hirsch I, Sproule S, Robb ML, Corey L, Neuzil KM, Hahn W, Hunt J, Mulligan MJ, et al. Phase 3 safety and efficacy of AZD1222 (ChAdOx1 nCoV-19) COVID-19 vaccine. N Engl J Med. 2021. https://doi.org/10.1056/NEJMoa2105290 .
El Sahly HM, Baden LR, Essink B, Doblecki-Lewis S, Martin JM, Anderson EJ, Campbell TB, Clark J, Jackson LA, Fichtenbaum CJ, et al. Efficacy of the mRNA-1273 SARS-CoV-2 vaccine at completion of blinded phase. N Engl J Med. 2021. https://doi.org/10.1056/NEJMoa2113017 .
Tanriover MD, Doğanay HL, Akova M, Güner HR, Azap A, Akhan S, Köse Ş, Erdinç F, Akalın EH, Tabak ÖF, et al. Efficacy and safety of an inactivated whole-virion SARS-CoV-2 vaccine (CoronaVac): interim results of a double-blind, randomised, placebo-controlled, phase 3 trial in Turkey. Lancet. 2021;398(10296):213–22. https://doi.org/10.1016/s0140-6736(21)01429-x .
Kumar S, Saurabh MK, Maharshi V. Efficacy and safety of potential vaccine candidates against coronavirus disease 2019: a systematic review. J Adv Pharm Technol Res. 2021;12(3):215–21. https://doi.org/10.4103/japtr.JAPTR_229_20 .
Burgos-Salcedo J. A rational strategy to support approved COVID-19 vaccines prioritization. Hum Vaccin Immunother. 2021;17(10):3474–7. https://doi.org/10.1080/21645515.2021.1922060 .
Download references
Acknowledgements
This study was funded by the National Natural Science Foundation of China (72122001; 71934002) and the National Science and Technology Key Projects on Prevention and Treatment of Major infectious disease of China (2020ZX10001002). The funders had no role in study design, data collection and analysis, decision to publish, or preparation of the paper. No payment was received by any of the co-authors for the preparation of this article.
Author information
Qiao Liu and Chenyuan Qin are joint first authors
Authors and Affiliations
Department of Epidemiology and Biostatistics, School of Public Health, Peking University, Beijing, 100191, China
Qiao Liu, Chenyuan Qin, Min Liu & Jue Liu
Institute for Global Health and Development, Peking University, Beijing, 100871, China
Chenyuan Qin & Jue Liu
You can also search for this author in PubMed Google Scholar
Contributions
LQ and QCY contributed equally as first authors. LJ and LM contributed equally as correspondence authors. LJ and LM conceived and designed the study; LQ, QCY and LJ carried out the literature searches, extracted the data, and assessed the study quality; LQ and QCY performed the statistical analysis and wrote the manuscript; LJ, LM, LQ and QCY revised the manuscript. All authors read and approved the final manuscript.
Corresponding authors
Correspondence to Min Liu or Jue Liu .
Ethics declarations
Ethics approval and consent to participate.
Not applicable.
Consent for publication
Competing interests.
The authors have no conflicts of interest to declare that are relevant to the content of this article.
Supplementary Information
Additional file 1: table s1..
Characteristic of studies included for vaccine effectiveness.
Additional file 2: Table S2.
Characteristic of studies included for vaccine safety.
Rights and permissions
Open Access This article is licensed under a Creative Commons Attribution 4.0 International License, which permits use, sharing, adaptation, distribution and reproduction in any medium or format, as long as you give appropriate credit to the original author(s) and the source, provide a link to the Creative Commons licence, and indicate if changes were made. The images or other third party material in this article are included in the article's Creative Commons licence, unless indicated otherwise in a credit line to the material. If material is not included in the article's Creative Commons licence and your intended use is not permitted by statutory regulation or exceeds the permitted use, you will need to obtain permission directly from the copyright holder. To view a copy of this licence, visit http://creativecommons.org/licenses/by/4.0/ . The Creative Commons Public Domain Dedication waiver ( http://creativecommons.org/publicdomain/zero/1.0/ ) applies to the data made available in this article, unless otherwise stated in a credit line to the data.
Reprints and permissions
About this article
Cite this article.
Liu, Q., Qin, C., Liu, M. et al. Effectiveness and safety of SARS-CoV-2 vaccine in real-world studies: a systematic review and meta-analysis. Infect Dis Poverty 10 , 132 (2021). https://doi.org/10.1186/s40249-021-00915-3
Download citation
Received : 07 September 2021
Accepted : 01 November 2021
Published : 14 November 2021
DOI : https://doi.org/10.1186/s40249-021-00915-3
Share this article
Anyone you share the following link with will be able to read this content:
Sorry, a shareable link is not currently available for this article.
Provided by the Springer Nature SharedIt content-sharing initiative
- Effectiveness
- Meta-analysis
Infectious Diseases of Poverty
ISSN: 2049-9957
- Submission enquiries: Access here and click Contact Us
- General enquiries: [email protected]
Comprehensive literature review on COVID-19 vaccines and role of SARS-CoV-2 variants in the pandemic
Affiliations.
- 1 School of Medicine, National University of Ireland, Galway, Ireland.
- 2 School of Medicine, National University of Ireland, Galway, University Road, Galway H91 TK33, Ireland.
- PMID: 34870090
- PMCID: PMC8637774
- DOI: 10.1177/25151355211059791
Since the outbreak of the COVID-19 pandemic, there has been a rapid expansion in vaccine research focusing on exploiting the novel discoveries on the pathophysiology, genomics, and molecular biology of the severe acute respiratory syndrome coronavirus 2 (SARS-CoV-2) infection. Although the current preventive measures are primarily socially distancing by maintaining a 1 m distance, it is supplemented using facial masks and other personal hygiene measures. However, the induction of vaccines as primary prevention is crucial to eradicating the disease to attempt restoration to normalcy. This literature review aims to describe the physiology of the vaccines and how the spike protein is used as a target to elicit an antibody-dependent immune response in humans. Furthermore, the overview, dosing strategies, efficacy, and side effects will be discussed for the notable vaccines: BioNTech/Pfizer, Moderna, AstraZeneca, Janssen, Gamaleya, and SinoVac. In addition, the development of other prominent COVID-19 vaccines will be highlighted alongside the sustainability of the vaccine-mediated immune response and current contraindications. As the research is rapidly expanding, we have looked at the association between pregnancy and COVID-19 vaccinations, in addition to the current reviews on the mixing of vaccines. Finally, the prominent emerging variants of concern are described, and the efficacy of the notable vaccines toward these variants has been summarized.
Keywords: AstraZeneca; BioNTech/Pfizer; COVID-19 vaccine; Moderna; SARS-CoV-2 variants.
© The Author(s), 2021.
Publication types
Click through the PLOS taxonomy to find articles in your field.
For more information about PLOS Subject Areas, click here .
Loading metrics
Open Access
Peer-reviewed
Research Article
COVID-19 and vaccine hesitancy: A longitudinal study
Roles Conceptualization, Data curation, Formal analysis, Investigation, Methodology, Project administration, Resources, Supervision, Validation, Visualization, Writing – original draft, Writing – review & editing
* E-mail: [email protected]
Affiliation Rady School of Management, University of California San Diego, La Jolla, California, United States of America

Roles Conceptualization, Data curation, Funding acquisition, Investigation, Methodology, Project administration, Supervision, Validation, Visualization, Writing – original draft, Writing – review & editing
Roles Conceptualization, Funding acquisition, Investigation, Methodology, Project administration, Supervision, Visualization, Writing – original draft, Writing – review & editing
- Ariel Fridman,
- Rachel Gershon,
- Ayelet Gneezy
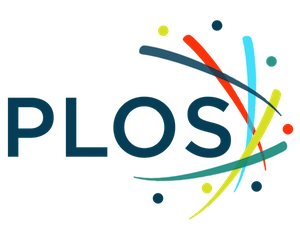
- Published: April 16, 2021
- https://doi.org/10.1371/journal.pone.0250123
- Peer Review
- Reader Comments
How do attitudes toward vaccination change over the course of a public health crisis? We report results from a longitudinal survey of United States residents during six months (March 16 –August 16, 2020) of the COVID-19 pandemic. Contrary to past research suggesting that the increased salience of a disease threat should improve attitudes toward vaccines, we observed a decrease in intentions of getting a COVID-19 vaccine when one becomes available. We further found a decline in general vaccine attitudes and intentions of getting the influenza vaccine. Analyses of heterogeneity indicated that this decline is driven by participants who identify as Republicans, who showed a negative trend in vaccine attitudes and intentions, whereas Democrats remained largely stable. Consistent with research on risk perception and behavior, those with less favorable attitudes toward a COVID-19 vaccination also perceived the virus to be less threatening. We provide suggestive evidence that differential exposure to media channels and social networks could explain the observed asymmetric polarization between self-identified Democrats and Republicans.
Citation: Fridman A, Gershon R, Gneezy A (2021) COVID-19 and vaccine hesitancy: A longitudinal study. PLoS ONE 16(4): e0250123. https://doi.org/10.1371/journal.pone.0250123
Editor: Valerio Capraro, Middlesex University, UNITED KINGDOM
Received: November 12, 2020; Accepted: February 14, 2021; Published: April 16, 2021
Copyright: © 2021 Fridman et al. This is an open access article distributed under the terms of the Creative Commons Attribution License , which permits unrestricted use, distribution, and reproduction in any medium, provided the original author and source are credited.
Data Availability: All data and code are publicly available on the Open Science Framework at https://osf.io/kgvdy/ .
Funding: UC San Diego Global Health Initiative (GHI): awarded to all authors; Project number: 1001288. The funders had no role in study design, data collection and analysis, decision to publish, or preparation of the manuscript. https://medschool.ucsd.edu/som/medicine/divisions/idgph/research/Global-Health/grant-recipients/2019-2020/Pages/Faculty-Postdoc-Travel-and-Research.aspx .
Competing interests: The authors have declared that no competing interests exist.
Introduction
Vaccinations are among the most important public health tools for reducing the spread and harm caused by dangerous diseases [ 1 ]. The World Health Organization estimates that vaccines prevented at least 10 million deaths between 2010–2015 worldwide [ 2 ]. Despite considerable evidence showing vaccines are safe [ 3 , 4 ], there is increasing skepticism toward vaccination [ 5 , 6 ]. Vaccine hesitancy has led to a decline in vaccine uptake and to an increase in the prevalence of vaccine-preventable diseases (VPDs) [ 7 , 8 ]. Ironically, the objection to vaccines is commonly a consequence of their effectiveness—because individuals have lower exposure to VPDs, they are less concerned about contracting them [ 9 ], which consequently leads to greater vaccine hesitancy [ 10 ]. The COVID-19 pandemic has created a new reality where individuals are faced with a previously unknown disease and its effects, providing a unique opportunity to investigate vaccine attitudes during a period of heightened disease salience. The present research reports findings from a longitudinal study conducted during the COVID-19 health crisis, in which we measured changes in attitudes toward a prospective vaccine, as well as shifts in vaccine attitudes in general.
Factors influencing vaccine attitudes and behaviors
Past research has identified a variety of situational and individual-level factors that influence vaccine attitudes and behavior, the most prominent of which are risk perceptions and demographic characteristics.
Assessments of risk are influenced by both cognitive evaluations (i.e., objective features of the situation such as probabilities of outcomes) and affective reactions [ 11 ], as well as by contextual factors (e.g., the information that is most available or salient at the time [ 12 ]). For example, research shows that media coverage plays a significant role in determining the extent to which we take threats seriously [ 13 ]. When individuals perceive heightened risk of a threat, they become more favorable toward interventions that mitigate that threat, including vaccination (for a meta-analysis on the effect of perceived risk on intentions and behaviors, see [ 14 ]). In the case of COVID-19, this would suggest more positive attitudes toward a vaccine and greater likelihood to get vaccinated. Indeed, research suggests that individuals should exhibit a greater interest in vaccinations during a pandemic because disease threat is more salient [ 15 ].
Past efforts to improve vaccine attitudes have had limited success or even backfired; for example, messages refuting claims about the link between vaccines and autism, as well as messages featuring images of children who were sick with VPDs, had negative effects on vaccine attitudes among those who were already hesitant to vaccinate [ 16 ]. In contrast, messaging that increases disease threat salience has shown promise in reducing vaccine hesitancy [ 5 ], and there is evidence suggesting that increased threat salience for a particular disease may also increase intentions to vaccinate for other diseases [ 17 ]. Building on these findings, we expected to find an increase in pro-vaccine attitudes and in individuals’ interest in a COVID-19 vaccine when the perceived threat of the COVID-19 virus increased.
Vaccine attitudes are also influenced by a variety of demographic and ideological factors (for a review, see [ 18 ]). For example, perceptions of vaccine risk differ among individuals of different ethnic backgrounds [ 19 ], and there is extant work demonstrating a positive correlation between socioeconomic status (SES) and vaccine hesitancy [ 20 , 21 ]. Socio-demographic factors are also linked to vaccine-related behaviors: among college students, those whose parents have attained a higher level of education are more likely to get immunized [ 22 ], and researchers have identified age as a predictor for receiving the influenza vaccine [ 23 ].
Political ideology is another well-documented determinant of vaccine-related attitudes and behaviors. Despite a common belief that liberals tend toward anti-vaccination attitudes in the United States, there is strong evidence that this trend is more present among conservatives [ 24 , 25 ]. According to a recent Gallup Poll, Republicans are twice as likely to believe the widely debunked myth that vaccines cause autism [ 26 ]. Recent work has shown that exposure to anti-vaccination tweets by President Trump—the first known U.S. president to publicly express anti-vaccination attitudes—has led to increased concern about vaccines among his supporters [ 27 ]. Based on these findings, and in conjunction with the sentiments expressed by the White House that diminished the significance of the pandemic [ 28 ], we expected to find diverging trends between Democrats and Republicans.
The current research
We collected vaccine-related attitudes of individuals living in the U.S. over a six-month period. Beginning in March 2020, we elicited attitudes from a cohort of the same individuals every month. We began data collection before any COVID-19 lockdown measures were in place (i.e., prior to the nation’s first shelter-in-place order [ 29 ]). Hence, our data spans the early phase of the pandemic, when there were fewer than 2,000 total confirmed cases in the U.S., through the following six months, at which point cumulative cases reached over 5.3 million [ 30 ].
Despite our prediction—that a public health crisis would increase disease threat, consequently increasing pro-vaccine attitudes and interest in vaccination—our data show an overall decrease in favorable attitudes toward vaccines. A closer look at the data revealed that political orientation explains more variance than any other socio-demographic variable. Specifically, participants who identify as Republican showed a decrease in their intention to get the COVID-19 vaccine and the influenza vaccine as well as a general decrease in pro-vaccine attitudes, whereas Democrats’ responses to these measures did not show a significant change during this period.
Our work is the first, to our knowledge, to longitudinally measure individuals’ attitudes toward vaccines. In doing so, our findings advance the understanding of how vaccine attitudes might change during an unprecedented public health crisis, revealing a strong association between political party affiliation and vaccine attitudes.
Participants
We recruited a panel of U.S. residents on Amazon’s Mechanical Turk platform to respond to multiple survey waves. To incentivize completion of all waves, we informed participants their payment would increase for subsequent surveys. Participants were paid 30 cents for wave 1, 40 cents for wave 2, and 60 cents for waves 3 and 4, $1.00 for wave 5, and $1.20 for wave 6. In addition, participants were informed that those who completed the first three waves would enter a $100 raffle. The median survey completion time was 5.5 minutes. The sample size for the first wave was 1,018, and the number of participants ranged from 608–762 on subsequent waves (see S1 Table for attrition details). This project was certified as exempt from IRB review by the University of California, San Diego Human Research Protections Program (Project #191273XX).
Our panel represents the broad and diverse population of the United States. The first wave sample included participants from all 50 states (except Wyoming) and Washington D.C., with an age range of 18 to 82 years old (mean = 38.48, median = 35). Approximately half (53%) identified as male, 46% as female, and.6% as other. The racial makeup in our sample was: 80% White, 9% Asian, 6% Black or African American, 4% multiple racial or ethnic identities, and 1% other. Relative to the U.S. Census (2019) [ 31 ] estimates, our sample over-represents White and Asian individuals, and under-represents Black or African American individuals and other racial groups.
We elicited political affiliation using a 6-point Likert scale, ranging from Strongly Republican to Strongly Democratic. In wave 1, 62% identified as Democrats and 38% identified as Republican, which is consistent with results from the most recent General Social Survey (GSS) [ 32 ]. There was no significant change in mean political identity from wave 1 to waves 2–6 (see S2 Table ). We classified participants as Democrats or Republicans using wave 1 political party affiliation. See S2 Appendix for additional details about the correlation of political party affiliation with age, gender, and SES.
Questions and measures
Our primary measure of interest was participants’ stated intention to get the COVID-19 vaccine when it becomes available. We were also interested in their perceptions of COVID-19 threat, general vaccination attitudes, and intention to get the flu shot. For all measures, except flu shot intentions, we combined multiple items to create a composite measure (see S2 Table for specific questions and construct compositions). Questions designed to measure general vaccination attitudes were adapted from prior work [ 33 ].
Additional measures of interest were participants’ trust in broad institutions (media, local government, and federal government). These trust measures followed different trends from each other, and therefore were not combined. At the end of the survey, participants responded to demographic questions. We retained all questions used in wave 1 throughout all six waves (our survey included additional items not reported in this paper; see S2 and S3 Tables for a complete list of measured items).
Data and analysis plan
Only participants with non-missing and non-duplicated responses were included in the analyses (see S1 Appendix for additional details). For all outcomes of interest, we tested for linear trends over time using a fixed effects regression specification [ 34 ]. All regression results include individual-level fixed effects, and standard errors are clustered at the individual level, to adjust for within-person correlation. We used this approach to control for the impact of omitted or unobserved time-invariant variables. P-values are not adjusted for multiple testing (see [ 35 ]). All analyses were conducted using R (version 4.0.2), and regressions were run using the package “fixest” (version 0.6.0). All materials, data, and additional analyses including robustness checks can be found here: https://osf.io/kgvdy/ .
We report results for three different vaccination-related measures: attitudes toward a COVID-19 vaccine, general vaccination attitudes, and flu shot intentions. All measures showed a decreasing trend (Ps < .001, except flu shot intentions where p = .05) for the 6-month duration of the study, indicating a reduction in pro-vaccination attitudes and intention to get vaccinated (COVID-19 and influenza vaccines). See S4 Table for full results of all regressions.
Heterogeneity in trend by political party
To better understand whether the decline in vaccine attitudes over time was driven by a particular factor, we used a data-driven approach, regressing all demographic characteristics on vaccine attitudes, in separate regressions. These demographics included education, income, SES, race, gender, an item measuring whether participants considered themselves to be a minority, whether the participant has children, and political party. Education, income, and SES were median split; race and gender were dummy coded; and political party affiliation was dichotomized into Democrat or Republican. Among all demographic characteristics, separating time trends by political affiliation (by adding an interaction term) attained the greatest adjusted within-R 2 in explaining vaccination attitude measures. In other words, political party affiliation explains the greatest within-individual variation in vaccine attitudes over time.
An analysis of responses by political affiliation revealed that the observed decreasing trend in all three vaccine measures was mostly driven by participants who identified as Republican (all Ps < .05), whereas Democrats’ responses showed either no significant trend (for COVID-19 vaccination and flu shot intentions: Ps >.67) or a significantly less negative time trend (general vaccination: p < .001). For these regressions, and moving forward, all results included interactions between wave and political party as well as interactions for wave and age, and wave and SES, to control for potentially different time trends associated with these variables. In each regression we also tested whether the strength of political affiliation moderates the observed results, and we reported the result when it did. We also conducted ANOVAs to compare mean responses for the outcomes of interest between Democrats and Republicans, separately for each wave (see S5 Table ).
COVID-19 vaccination attitudes ( Fig 1 , Panel A).
- PPT PowerPoint slide
- PNG larger image
- TIFF original image
Points represent means, and error bars represent 95% confidence intervals. All scale responses range from 1 to 7.
https://doi.org/10.1371/journal.pone.0250123.g001
A two-item construct ( r = .78) was created, with greater values corresponding to more favorable responses.
In wave 1, Democrats ( M = 5.39, SD = 1.55) had more favorable attitudes toward a COVID-19 vaccine than Republicans ( M = 4.57, SD = 1.76; t = -7.38, p < .001, d = -.48, 95% CI = [-.61, -.35]). Among Democrats, there was no significant time trend ( β = .02, SE = .04, p >.67) whereas Republicans’ responses followed a decreasing time trend ( β = -.09, SE = .05, p = .046). These trends were significantly different from each other ( β = -.11, SE = .02, p < .001).
General vaccination attitudes ( Fig 1 , Panel B).
A ten-item construct ( α = .95) was created, with greater values corresponding to a more positive attitude toward vaccination in general.
In wave 1, Democrats ( M = 5.83, SD = 1.15) expressed more favorable general vaccination attitudes than Republicans ( M = 5.17, SD = 1.31; t = -7.91, p < .001, d = -.52, 95% CI = [-.66, -.39]). Although both Democrats and Republicans had a decreasing time trend (Democrats: β = -.04, SE = .02, p = .029; Republicans: β = -.09, SE = .02, p < .001), the trend for Republicans was significantly more negative ( β = -.04, SE = .01, p < .001).
Flu shot intentions ( Fig 1 , Panel C).
We asked participants whether they plan to get the flu shot next year, with greater values indicating greater intentions.
In wave 1, Democrats ( M = 4.84, SD = 2.34) indicated greater intentions to vaccinate against the flu than Republicans ( M = 4.35, SD = 2.39; t = -3.15, p = .002, d = -.21, 95% CI = [-.34, -.08]). Among Democrats, there was no significant time trend ( β = .01, SE = .04, p = .86), suggesting their vaccination intentions remained largely stable. Republicans’ responses, however, revealed a decreasing time trend ( β = -.12, SE = .04, p = .005), and the two trends were significantly different from each other ( β = -.12, SE = .02, p < .001).
Our analyses revealed an interaction with political affiliation strength among Republicans, whereby participants who identified as more strongly Republican had a more negative time trend ( β = -.05, SE = .02, p = .027). This interaction was not significant for Democrats ( β = -.02, SE = .01, p = .19).
Perceived threat of COVID-19 ( Fig 2 ).
https://doi.org/10.1371/journal.pone.0250123.g002
A three-item construct ( α = .82) was created, with greater perceived threat about COVID-19.
In wave 1, Democrats ( M = 4.26, SD = 1.25) expressed greater perceived threat of COVID-19 than Republicans ( M = 3.90, SD = 1.39; t = -4.14, p < .001, d = -.40, 95% CI = [-.27, -.14]). Democrats’ responses showed an increasing time trend ( β = .08, SE = .04, p = .033), indicating they became increasingly concerned about the threat posed by the virus over time. Among Republicans, there was no significant time trend ( β = -.01, SE = .04, p = .83). These trends were significantly different from each other ( β = -.09, SE = .02, p < .001). While our data does not render causal claims, it is possible that the divergence in COVID-19 threat perceptions over time among Republicans and Democrats contributes to the divergence in vaccine attitudes between these groups over time. We revisit this proposition in the General Discussion.
Our analyses revealed an interaction with political affiliation strength among Democrats—participants who identified as more strongly Democrat had a more positive time trend ( β = .03, SE = .01, p = .019), suggesting an increasing threat perception over time. This interaction was not significant for Republicans ( β = .01, SE = .02, p = .61).
Trust in broad institutions.
The measures of trust in media, local government, and federal government were not highly correlated ( α = .66), and were therefore analyzed separately.
Trust in media ( Fig 3 , Panel A) . In wave 1, Democrats ( M = 3.61, SD = 1.66) reported greater trust in the media than Republicans ( M = 2.73, SD = 1.65; t = -8.12, p < .001, d = -.53, 95% CI = [-.66, -.39]). There was no significant time trend for either Democrats ( β = .02, SE = .04, p = .57) or Republicans ( β = -.05, SE = .04, p = .20). However, the trend for Republicans was significantly more negative ( β = -.07, SE = .02, p < .001). The different trends we observe for Democrats and Republicans with respect to trust in the media may explain the divergence in perceived threat and vaccine attitudes between these groups over time (see General discussion ).
https://doi.org/10.1371/journal.pone.0250123.g003
Trust in local government ( Fig 3 , Panel B) . In wave 1, Democrats ( M = 4.07, SD = 1.60) indicated lower trust in local government than Republicans ( M = 4.28, SD = 1.60; t = 2.01, p = .045, d = .13, 95% CI = [.003,.26]). Among Democrats, there was no significant time trend ( β = -.06, SE = .04, p = .18), though among Republicans, there was a decreasing time trend ( β = -.11, SE = .05, p = .015). These trends were significantly different from each other ( β = -.06, SE = .02, p = .004).
Trust in federal government ( Fig 3 , Panel C) . In wave 1, Democrats ( M = 2.96, SD = 1.67) expressed lower trust in the federal government than Republicans ( M = 4.08, SD = 1.60; t = 10.52, p < .001, d = .68, 95% CI = [.55,.82]). Both Democrats and Republicans had decreasing time trends (Democrats: β = -.08, SE = .04, p = .036; Republicans: β = -.10, SE = .04, p = .025). These trends were not significantly different from each other ( β = -.02, SE = .02, p = .37).
To rule out differential attrition, we tested whether the composition of our sample (i.e., age, gender, and political party) changed over time (see S1 Table ). Specifically, we tested whether participants who responded to waves 2–6 were significantly different at baseline (wave 1) from the full sample at baseline. The only significant change detected (Ps < .05) was with respect to participants’ age, though the differences were small—the average age was 38.5 at baseline, and remained between 39.9 and 40.8 at baseline among participants who responded to subsequent waves. We found no other systematic pattern of attrition among our participants.
General discussion
Over the course of six months of the COVID-19 pandemic, beginning with a relatively early phase prior to any U.S. directives to stay home (March 2020) and continuing through a cumulation of over 5 million cases (August 2020), we found a decrease in pro-vaccine attitudes and COVID-19 vaccination intentions, as well as reduced intentions to get the influenza vaccine. These findings are contrary to our prediction that increased salience of COVID-19 would improve attitudes toward vaccines.
Our analyses identify political ideology as the best predictor of the decreasing time trend across our three vaccine-related attitudes and intentions measures. In particular, we found that while Democrats’ responses remained fairly stable over time, Republicans shifted away from their lower initial responses and from Democrats’ responses, leading to increased polarization throughout the six-month period.
Contrary to the polarization observed in our data, social and behavioral scientists have long argued that groups facing threats often come together, demonstrating stronger social cohesion [ 36 ], and more cooperative behaviors [ 37 , 38 ]. Researchers have also found that individuals’ sense of shared identity plays a role in promoting cooperative behavior in response to threat [ 39 – 41 ]. Considering our results in the context of these findings might suggest that our respondents’ sense of shared identity was dominated by their political ideology, as opposed to a broader (e.g. American) identity.
What might be going on?
Although the nature of our data does not render causal claims, it highlights potential explanations. First, we note that participants’ ratings of perceived COVID-19 threat followed a similar diverging pattern by party affiliation to our three vaccine-related measures during the study period. Democrats perceived COVID-19 threat to be greater at the start of the study than Republicans did, and this gap widened significantly as the study progressed. This trend is consistent with previous research showing that vaccine hesitancy is related to perceived risk of a threat; when a VPD threat level is low, individuals are less motivated to take preventative action (i.e., immunize; for a review, see [ 42 ]).
Our data offers one potential explanation for the polarization of threat perception: Republican and Democratic participants in our study reported consuming different sources of information. The most commonly checked news source for Republicans was Fox News (Republicans: 50%, Democrats: 8%; χ 2 = 164.55, p < .001) and for Democrats was CNN (Democrats: 47%, Republicans: 23%, χ 2 = 43.08, p < .001, see S6 Table ). Corroborating this proposition, a Pew Research Center poll conducted in March 2020 found that 56% of respondents whose main news source is Fox News believed that “the news media have greatly exaggerated the risks about the Coronavirus outbreak,” whereas this was only true for 25% of those whose main news source is CNN [ 43 ]. Of note, Facebook and Instagram, were also in the top four most consumed news sources for participants affiliated with either party. Extant work describes these platforms as echo chambers [ 44 , 45 ], which may exacerbate partisan exposure to news and information.
Another trend highlighted by our data shows that similar to vaccine attitudes, Republicans’ trust in the media decreased significantly more during our study than Democrats’, suggesting these patterns might be related. According to Dr. Heidi Larson, an expert on vaccine hesitancy and founder of the Vaccine Confidence Project, misinformation regarding vaccinations is more likely to take root when individuals do not trust the information source [ 46 ]. Future research might further examine the role of trust in the media on vaccine attitudes.
While trust in media or media exposure may be driving COVID-19 threat perceptions and vaccine attitudes, there are many other possible explanatory factors that are not captured by our data or analyses. For example, it is possible that threat perceptions were influenced by how a respondents’ county or state was affected by COVID-19; up until June 2020, COVID-19 cases were more common in Democrat-leaning states [ 47 ], which might have amplified its salience early on and influenced attitudes and behavior. Further, although we included individual-level fixed-effects which control for all time invariant participant characteristics, and controlled for different trends by age and SES, we cannot rule out the possibility that other factors (e.g., educational attainment or population density) may have influenced the observed trends. Finally, as our data collection began after the onset of COVID-19, it is possible that the trend we observe for Republicans represents a return to a pre-pandemic baseline of vaccine-related attitudes.
Contributions
This work advances our understanding of how health-related attitudes evolve over time. Our focus on vaccine-related attitudes and intentions is important because experts agree that having enough people vaccinate against COVID-19 is key to stemming the pandemic [ 48 ]. More broadly, negative attitudes toward vaccination in general, and reduced vaccine uptake, is increasingly a public health concern [ 49 ]. This research provides insight into the trends of such vaccine hesitancy, underlining the importance of risk salience and its roots in ideology and media exposure.
This work also contributes to our understanding of political parties and polarization. Numerous anecdotes and reports have demonstrated a partisan divide in Americans’ response to the COVID-19 pandemic. For example, research found greater negative affective responses to wearing a face covering among politically right (vs. left) leaning individuals [ 50 ]. Here, we show that although these observations are valid, the reality is more nuanced. For example, our analyses reveal that polarization on vaccine measures—both attitudes and intentions—is driven primarily by self-identified Republicans’ gradual movement away from their initial responses whereas Democrats’ responses remained largely stable. This insight has important practical implications: It informs us about the dynamics of individuals’ attitudes, bringing us closer to understanding the underlying factors that influence attitudes and behaviors. Equipped with this knowledge, one could design more effective communications and interventions.
Note on methodology and data availability
The present study contributes to a small but growing literature in the social sciences using longitudinal data [ 51 ]. Using a longitudinal methodology allowed us to track individual-level changes over time. Merely observing a single point in time would allow us to observe across-group differences, but would lack the bigger picture of how polarization between these groups evolved. Another key advantage of panel data is that it allows us to include individual-level fixed effects, which control for the impact of omitted or unobserved time-invariant variables. Finally, panel data allows for more accurate inference of model parameters [ 52 ].
While the focus of this paper is vaccine attitudes, our broad dataset offers a unique opportunity to understand attitudes and behavior over time. Due to the richness of our data, its unique nature, and its timeliness, we believe it is important to make it available to other researchers interested in exploring it and publishing additional findings. The complete dataset is available at https://osf.io/kgvdy/ (see S2 and S3 Tables for all items collected).
Supporting information
S1 appendix. additional information about sample exclusions..
https://doi.org/10.1371/journal.pone.0250123.s001
S2 Appendix. Additional information about political party affiliation.
https://doi.org/10.1371/journal.pone.0250123.s002
S1 Table. Attrition table.
https://doi.org/10.1371/journal.pone.0250123.s003
S2 Table. Summary table of measures and constructs included in the text.
https://doi.org/10.1371/journal.pone.0250123.s004
S3 Table. Summary table of measures excluded from the text.
https://doi.org/10.1371/journal.pone.0250123.s005
S4 Table. Regression results.
https://doi.org/10.1371/journal.pone.0250123.s006
S5 Table. Outcome measures by political party affiliation.
https://doi.org/10.1371/journal.pone.0250123.s007
S6 Table. Summary of news sources.
https://doi.org/10.1371/journal.pone.0250123.s008
- View Article
- Google Scholar
- 2. World Health Organization. The Power of Vaccines: Still not fully utilized. WHO; 2020. https://www.who.int/publications/10-year-review/vaccines/en/ .
- 4. Immunization Safety Review Committee. Immunization safety review: vaccines and autism. National Academies Press; 2004 Sep 30.
- PubMed/NCBI
- 25. Luton R, Hare C. Conservatives are more likely to believe that vaccines cause autism. The Washington Post. 2015 March 1. https://www.washingtonpost.com/news/monkey-cage/wp/2015/03/01/conservatives-are-more-likely-to-believe-that-vaccines-cause-autism/ .
- 26. Reinhart R. Fewer in US continue to see vaccines as important. 2020 Jan 24. https://news.gallup.com/poll/276929/fewer-continue-vaccines-important.aspx .
- 28. Summers, Juana Timeline: How Trump Has Downplayed The Coronavirus Pandemic. NPR. 2020 October 2, 2020. https://www.npr.org/sections/latest-updates-trump-covid-19-results/2020/10/02/919432383/how-trump-has-downplayed-the-coronavirus-pandemic
- 29. Ortiz J, Hauck G. Coronavirus in the US. USA Today. 2020 March 30, 2020. https://www.usatoday.com/story/news/nation/2020/03/30/coronavirus-stay-home-shelter-in-place-orders-by-state/5092413002/ .
- 30. Elflein J. State of Health. World Health Organization. 2020 October 5. https://www.statista.com/statistics/1103185/cumulative-coronavirus-covid19-cases-number-us-by-day/ .
- 31. United States Census Bureau. Quick Facts. 2019. https://www.census.gov/quickfacts/fact/table/US/PST045219 .
- 32. Smith TW, Davern M, Freese J, Morgan S. General Social Surveys, National Science Foundation. 2020. gssdataexplorer.norc.org
- 34. Wooldridge JM. Econometric analysis of cross section and panel data. MIT press; 2010 Oct 1.
- 46. Anderson J. She Hunts Viral Rumors about Real Viruses. The New York Times. 2020 October 13. https://www.nytimes.com/2020/10/13/health/coronavirus-vaccine-hesitancy-larson.html .
- 47. Bump P. Coronavirus has come to Trump country. The Washington Post. 2020 June 17. https://www.washingtonpost.com/politics/2020/06/17/coronavirus-has-come-trump-country/ .
- 48. Quinn M. Fauci warns U.S. "unlikely" to reach Herd Immunity if too Many Refuse Vaccine. CBS News. 2020 June 29. https://www.cbsnews.com/news/fauci-herd-immunity-coronavirus-vaccine/ .
- 49. World Health Organization. Improving vaccination demand and addressing hesitancy. WHO; 2019. [cited 2020 November 3]. https://www.who.int/immunization/programmes_systems/vaccine_hesitancy/en/ .
Thesis Helpers

Find the best tips and advice to improve your writing. Or, have a top expert write your paper.
170 Vaccination Research Paper Topics For Stellar Students

Research papers are a monumental highlight in your academic journey. They are a critical milestone in your studies that must be tackled with the utmost care and stellar diligence. Vaccination topics are susceptible as you have to show complete mastery of all details.
If you are pursuing a medicine course, then vaccination research topics might be an excellent area of interest. A good research paper starts with a great topic, and we are here to help you nail that. We understand the significance of research papers, and that is why we have handpicked 170 out-of-the-box vaccination research paper topics, titles, and ideas to make your work seamless.
Debate Topics About Vaccination
- What is reverse vaccinology?
- Look at the ways of harnessing the participation of dendritic cells in tolerance and immunity
- What are some of the approaches to advance cancer vaccines to clinical utility?
- Highlight innovative therapeutic and vaccine approaches against respiratory pathogens
- Examine immunity to malaria and vaccine strategies
- Assess molecular vaccines against pathogens in the post-genomic era
- Comprehending the limitations of today’s influenza vaccine strategies and further development of more efficient therapeutic and preventative interventions
- Study HIV-associated persistent inflammation and immune activation
- Analyze recent advances in respiratory virus infection
- What is the novel approach for anti-tumor vaccines
- Unravel the challenges and progress in the development of a B cell-based hepatitis C virus vaccine
- What is the functional relevance of Tatraspanins in the immune system?
- Look at advanced immunization technologies for next-generation vaccines
- Evaluate epitope discovery and synthetic vaccine design
- In what ways can tuberculosis be treated by targeting host immunity
- What are the immunomodulatory effects of drugs in the treatment of immune-related diseases
- Highlight natural antibodies in health and disease
- Discuss different influenza virus vaccines and immunotherapy
- What are some of the shadows of cancer immunotherapy
- Understanding the therapeutical potential of extracellular vesicles
- A review of the ethical theories and problems associated with vaccination in America
- Do vaccines love the Darwinian fitness of immune cells
Vaccination Behavior Research Topics
- Unraveling demand and supply effects on the up-take of influenza vaccinations
- Point out new approaches to the seasonal flu vaccine
- Exploring the impact of vaccination
- Investigating patient experience with, and the use of, an electronic monitoring system to assess vaccination responses
- A meta-analysis of interventions that enhance the use of adult immunization and cancer screening services
- Do vaccines seem to work against bacterial and viral infections, and are they effective?
- Gathering the evidence for the introduction of typhoid vaccine: worldwide vaccine testing
- Explore molecular mimicry to broaden the immune response to carbohydrate antigens for vaccine development
- Tumor-associated glycan and immune surveillance
- Rational design and application of idiotope vaccines
- Assessing the effects of vaccines on immune-deficient people
- What are the impacts of rapid growth and deployment of high-volume vaccines for pandemic response
Anti-vaccination Research Paper Topics
- Should the state impose vaccinations, or should the choice be left up to the child’s parents?
- What is the connection between vaccination and autism?
- Is natural immunity better than immunity through immunization?
- Examining cultural perspectives on vaccination
- Are they worth it? adverse effects of vaccination on children
- To vaccinate or not against HPV? A content analysis of vocabularies of motives
- Vaccines: religious and cultural arguments from an Islamic perspective
- Anti-science populism or biomedicine’s unresolved knots? Comparing views on the movements against mandatory pediatric vaccines
- An anthropological commentary on vaccine hesitancy, decision-making, and interventionism among religious minorities
- Understanding attitudes to vaccination
Research Topics For Covid-19 Vaccination
- Medical mistrust in the context of Covid-19: implications for intended care-seeking and quarantine policy support in the United States
- What is the acceptability of the potential COVID-19 vaccine among smokers and non-smokers?
- COVID-19 vaccine hesitancy in healthcare personnel: are there any differences among classifications
- Discuss various options that one can use to convince people to get the covid-19 vaccine
- Examining COVID-19 vaccine efficacy after the first dose: Pfizer, Moderna, AstraZeneca
- Discuss the impacts of herd immunity during the covid-19 pandemic
- What are some of the effects of covid-19 vaccination on transmission of disease?
- Discuss whether antibodies generated through vaccination recognize all-new variants of covid-19
- Investigate how the intensity of lockdowns accelerate or influence mutation of the COVID virus
- Examine how the new covid-19 strain identified in England will affect the available vaccines.
- Outline which immunoglobulin types can be used as the markers for covid-19 vaccination
- Which is the best way to deal with swaps after completing vaccinations in nursing homes
- How do we curb vaccine hesitancy among healthcare providers?
- Which one is the more dangerous, covid-19 or covid-19 vaccine? What must be the individual decision?
- Analyzing Ebola and the evolving ethics of quarantine
- Break down some of the side effects of covid-19 vaccination
- How long will immunity last after receiving the covid-19 vaccination?
- Will, a covid-19 vaccine work for everyone? Are there people who cannot get vaccinated?
- Is bivalent OPV immunization capable of mitigating the impact of covid-19?
- What are the expected long-term side effects of the vaccination for covid-19?
- Evaluate differences between the first and second doses of the covid-19 mRNA vaccine?
- Examine the ingredients in the covid-19 mRNA vaccine
- Can a person’s DNA change through mRNA vaccines?
- Factors that stops the body from continuing to produce COVID-19 spike protein after getting a COVID-19 mRNA
- Discuss whether a person vaccinated against covid-19 will be able to spread the virus to susceptible people
- Investigating vaccination adverse outcomes and costs of vaccine injury claims(VICs): In the past, present, and during COVID-19.
- Who gets cured: Covid-19 and the development of critical sociology and anthropology of cure
- Development of perception and attitude scales related to COVID-19 pandemic
- Does the mutation of the coronavirus affect the capacity of the vaccines to prevent disease?
- A case-control study: finding a link between pre-existing antibodies got after the childhood vaccinations or past infections and COVID-19?
- Queue questions: ethics of COVID-19 vaccine prioritization
- Disparities between Black and White in H1N1 vaccination among adults in the U.S. in 2009: A cautionary tale for the COVID-19 pandemic
- Autonomy and refusal in pandemics: What to do with those who refuse COVID-19 vaccines
- Knowledge, attitude, and acceptance of a COVID-19 vaccine: a global cross-sectional study
- Prospects of COVID-19 vaccine implementation in the U.S.: Challenges and potential solutions
- What are the effects of COVID-19 vaccines on pregnant women?
- Compare and contrast the efficacy of different covid-19 vaccines.
- Ways to improve covid-19 vaccine acceptance
- Determination of causation between COVID-19 vaccines and potential adverse effects
Vaccination Of Children Topics
- What is the essence of increasing HPV vaccination among children?
- Analyze the primary diseases that vaccines prevent in children
- What will happen if a child’s vaccination schedule is delayed
- Look at the vaccination schedule for children in the U.S.
- Can children receive more than one vaccine at a time?
- Examine revaccination outcomes of children with proximate vaccine seizures
- What are the impacts of measles-containing vaccination in children with the severe underlying neurologic disease?
- Evaluate the challenges involved in measuring immunization activity coverage among measles zero-dose children
- What is the connection between the polio vaccine and the risk of cancer among children?
- Do multiple vaccines affect babies’ health and immune system in an adverse war, or can their bodies handle them?
- What are the various vaccination options available for children, and are they harmful to children’s overall health?
- The case for further research and development: assessing the potential cost-effectiveness of microneedle patches in childhood measles vaccination programs
- Evaluate the accuracy of parental recall of child immunization in an inner-city population
- Evaluating maternal acculturation and childhood immunization levels among children in African-American families in Florida
- Policy analysis: the impact of the vaccine for children’s program on child immunization delivery
- The effect of managed care: investigating access of infant immunizations for poor inner-city families
- Who takes up free flu shots? Investigating the effects of an expansion in coverage
- What are the societal and parental values for the risks and benefits of childhood combination vaccines?
- Looking into trends in vaccination intentions and risk perceptions: a longitudinal study of the first year of the H1N1 pandemic
Healthcare Topics About Vaccination
- Conscious consideration of herd immunity in influenza vaccination decisions
- A case study of ethnic or racial differences in Medicare experiences and immunization
- What preservatives are used in vaccines
- Discuss the relationship between vaccines and autism
- What is the role of epidemiology in infection control?
- How t design and select the most relevant immunogenic peptide sequences
- Discuss why the Zika virus has not had a significant impact in Africa as compared to America
- What are the advantages of using the phage display technology of antibodies versus hybridism technology?
- Analyzing the impact and cost-effectiveness of vaccination programs in a country using mathematical models
- Malaria vaccines: progress and problems
- Malaria: cloning genes for antigens of plasmodium falciparum
- Fighting profits on the pandemic: The fight for vaccines in today’s economic and geopolitical context
- Molecular and biotechnological approaches to fish vaccines
- Immunogenicity of a whole-cell pertussis vaccine with low lipopolysaccharide content in infants
- Immunogrid: an integrative environment for large-scale simulation of the immune system for vaccine discovery, design, and optimization
Thesis Topics In Vaccination
- Investigating challenges and opportunities in vaccine delivery, discovery, and development
- Discuss classic methods of vaccine development
- What are some of the current problems in vaccinology?
- Assess some of the latest tools for vaccine development
- Using cost-effectiveness analysis to support research and development portfolio prioritization for product innovations in measles vaccination
- Communicating vaccine safety during the introduction and development of vaccines
- Highlighting viral vectors for use in the development of biodefense vaccines
- What is the role of US. military research programs in the invention of USA-approved vaccines for naturally occurring infectious diseases
- Curbing outbreaks: utilizing international governmental risk pools to fund research and development of infectious disease medicines and vaccines
- Vaccine stabilization: research commercialization and likely impacts
- Exam the unequal interactions of the role of patient-centered care in the inequitable diffusion of medical innovation, the human papillomavirus(HPV) vaccine
- A case study of the status of development of vaccines and vaccine research for malaria
- Enteric infections vs vaccines: a public health and clinical research agenda for developing countries
- A review of research and vaccine development for industry animals in third world countries
- How the research-based industry approaches vaccine development and establishes priorities
- A look at the status of vaccine research and development of a vaccine for HIV-1
- Modeling a cost-effective vaccination strategy for the prevention of herpes zoster infection
- Using an adequate T.B. vaccination regiment to identify immune responses associated with protection in the murine model
- A systematic analysis of the link between vaccines and atopic dermatitis
- Do vaccines provide better immunity than natural infections?
- Is there a need to be vaccinated against a disease that is not available in your country or community
- How to strengthen adult immunization via coordinated action
- Using the general equilibrium method to assess the value of a malaria vaccine: An application to African countries
- Who should take up free flu shots?
- Evaluate the impact of vaccination among health care personnel
- Retail clinics and their impact on vaccination in the U.S.
- Discuss the societal values for the benefits and risks of childhood combination vaccines
- How safe and effective is the synovial vaccine for people above 60 years
- Evaluating vaccination effectiveness of group-specific fractional-dose strategies
Law Research Topics On Vaccination
- Explain why there are age restrictions for Rotavirus vaccination?
- Vaccination or hygiene : Which factor contributes to the decline of infectious diseases?
- Outline the main factors that cause vaccine failure
- Discuss why HIV is so hard to vaccinate in uninfected people?
- In what ways do maternal vaccinations affect the fetal nervous system development
- How to deliver malaria vaccine effectively and efficiently
- Highlight the vaccines that are specifically licensed in the U.S. for pregnant women
- How does an immune genetic algorithm work?
- Evaluate the relationship between the success of artificial insemination and vaccination
- Outline the reasons why vaccines underperform in low-income countries
- Discuss U.S. immigration and vaccination policy
- Assessing the effectiveness of compelled vaccination
Vaccination Ethical Topics
- What are the requirements for a strain to be used as a vaccine?
- What is the best way to administer vaccines in children?
- Assessing the benefits of maternal vaccination on breastfed infants
- Evaluating the pros and cons of intraperitoneal vaccination
- Examine ways to measure the pattern of vaccination acceptance
- Investigate Covid-19 transmission, vaccination rate, and the fate of resistant strains
- Look into dark web marketplaces and covid-19 vaccines.
- A close look at covid-19 vaccines and kidney diseases
- Contextualizing the impact of covid-19 vaccine misinformation on vaccination intent in the U.S.
- Examining behaviors and attitudes of medical students towards covid-19 vaccines
From the exceptional vaccination debate topics, titles, and other ideas above, you can quickly tell that we have your best interest at heart. We believe that all students deserve decent scores on their papers. As a result, we are more than willing to recommend affordable online help with research papers from the best-rated sites.
Whether you are overwhelmed by other assignments, dealing with a tight deadline, or lack the spark to pull it off, you can count on cheap yet professional thesis help from trusted assignment help sites. They are ready to deliver high-quality papers, and you can confirm that from any sample or example on the platforms.

Make PhD experience your own
Leave a Reply Cancel reply
Your email address will not be published. Required fields are marked *
Thank you for visiting nature.com. You are using a browser version with limited support for CSS. To obtain the best experience, we recommend you use a more up to date browser (or turn off compatibility mode in Internet Explorer). In the meantime, to ensure continued support, we are displaying the site without styles and JavaScript.
- View all journals
- Explore content
- About the journal
- Publish with us
- Sign up for alerts
- Review Article
- Published: 22 December 2020
A guide to vaccinology: from basic principles to new developments
- Andrew J. Pollard ORCID: orcid.org/0000-0001-7361-719X 1 , 2 &
- Else M. Bijker 1 , 2
Nature Reviews Immunology volume 21 , pages 83–100 ( 2021 ) Cite this article
490k Accesses
686 Citations
3673 Altmetric
Metrics details
- Infectious diseases
A Publisher Correction to this article was published on 05 January 2021
This article has been updated
Immunization is a cornerstone of public health policy and is demonstrably highly cost-effective when used to protect child health. Although it could be argued that immunology has not thus far contributed much to vaccine development, in that most of the vaccines we use today were developed and tested empirically, it is clear that there are major challenges ahead to develop new vaccines for difficult-to-target pathogens, for which we urgently need a better understanding of protective immunity. Moreover, recognition of the huge potential and challenges for vaccines to control disease outbreaks and protect the older population, together with the availability of an array of new technologies, make it the perfect time for immunologists to be involved in designing the next generation of powerful immunogens. This Review provides an introductory overview of vaccines, immunization and related issues and thereby aims to inform a broad scientific audience about the underlying immunological concepts.

Similar content being viewed by others
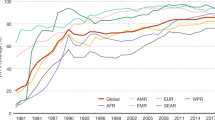
Immunization: vital progress, unfinished agenda
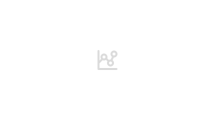
The non-specific and sex-differential effects of vaccines
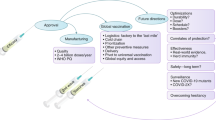
Looking beyond COVID-19 vaccine phase 3 trials
Introduction.
Vaccines have transformed public health, particularly since national programmes for immunization first became properly established and coordinated in the 1960s. In countries with high vaccine programme coverage, many of the diseases that were previously responsible for the majority of childhood deaths have essentially disappeared 1 (Fig. 1 ). The World Health Organization (WHO) estimates that 2–3 million lives are saved each year by current immunization programmes, contributing to the marked reduction in mortality of children less than 5 years of age globally from 93 deaths per 1,000 live births in 1990 to 39 deaths per 1,000 live births in 2018 (ref. 2 ).
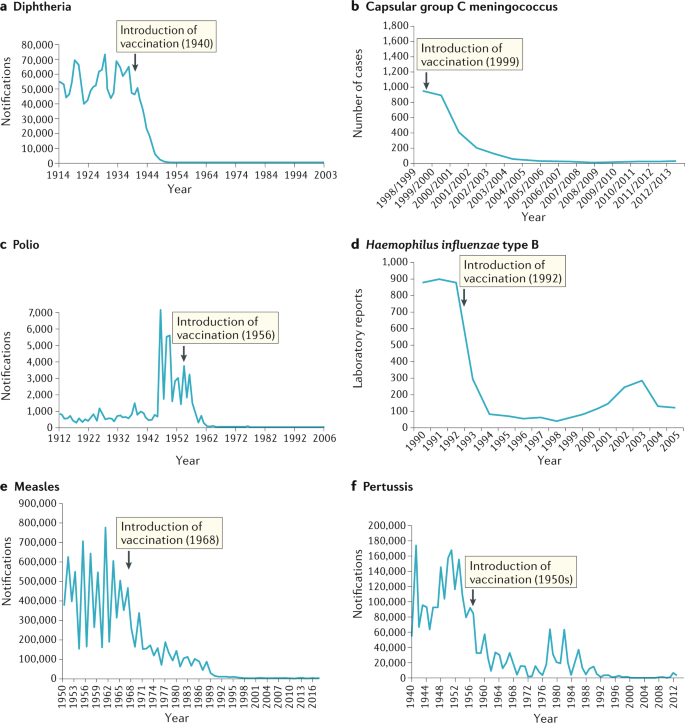
The introduction of vaccination against infectious diseases such as diphtheria (part a ), capsular group C meningococcus (part b ), polio (part c ), Haemophilus influenzae type B (part d ), measles (part e ) and pertussis (part f ) led to a marked decrease in their incidence. Of note, the increase in reports of H. influenzae type B in 2001 led to a catch-up vaccination campaign, after which the incidence reduced. For pertussis, a decline in vaccine coverage led to an increase in cases in the late 1970s and 1980s, but disease incidence reduced again after vaccine coverage increased. Adapted with permission from the Green Book, information for public health professionals on immunisation, Public Health England , contains public sector information licensed under the Open Government Licence v3.0.
Vaccines exploit the extraordinary ability of the highly evolved human immune system to respond to, and remember, encounters with pathogen antigens . However, for much of history, vaccines have been developed through empirical research without the involvement of immunologists. There is a great need today for improved understanding of the immunological basis for vaccination to develop vaccines for hard-to-target pathogens (such as Mycobacterium tuberculosis , the bacterium that causes tuberculosis (TB)) 3 and antigenically variable pathogens (such as HIV) 4 , to control outbreaks that threaten global health security (such as COVID-19 or Ebola) 5 , 6 and to work out how to revive immune responses in the ageing immune system 7 to protect the growing population of older adults from infectious diseases.
In this Review, which is primarily aimed at a broad scientific audience, we provide a guide to the history (Box 1 ), development, immunological basis and remarkable impact of vaccines and immunization programmes on infectious diseases to provide insight into the key issues facing immunologists today. We also provide some perspectives on current and future challenges in continuing to protect the world’s population from common pathogens and emerging infectious threats. Communicating effectively about the science of vaccination to a sceptical public is a challenge for all those engaged in vaccine immunobiology but is urgently needed to realign the dialogue and ensure public health 8 . This can only be achieved by being transparent about what we know and do not know, and by considering the strategies to overcome our existing knowledge gaps.
Box 1 A brief history of vaccination
Epidemics of smallpox swept across Europe in the seventeenth and eighteenth centuries, accounting for as much as 29% of the death rate of children in London 137 . Initial efforts to control the disease led to the practice of variolation, which was introduced to England by Lady Mary Wortley Montagu in 1722, having been used in the Far East since the mid-1500s (see Nature Milestones in Vaccines ). In variolation, material from the scabs of smallpox lesions was scratched into the skin in an attempt to provide protection against the disease. Variolation did seem to induce protection, reducing the attack rate during epidemics, but sadly some of those who were variolated developed the disease and sometimes even died. It was in this context that Edward Jenner wrote ‘An Inquiry into the Causes and Effects of the Variole Vaccinae…’ in 1798. His demonstration, undertaken by scratching material from cowpox lesions taken from the hands of a milkmaid, Sarah Nelms, into the skin of an 8-year-old boy, James Phipps, who he subsequently challenged with smallpox, provided early evidence that vaccination could work. Jenner’s contribution to medicine was thus not the technique of inoculation but his startling observation that milkmaids who had had mild cowpox infections did not contract smallpox, and the serendipitous assumption that material from cowpox lesions might immunize against smallpox. Furthermore, Jenner brilliantly predicted that vaccination could lead to the eradication of smallpox; in 1980, the World Health Assembly declared the world free of naturally occurring smallpox.
Almost 100 years after Jenner, the work of Louis Pasteur on rabies vaccine in the 1880s heralded the beginning of a frenetic period of development of new vaccines, so that by the middle of the twentieth century, vaccines for many different diseases (such as diphtheria, pertussis and typhoid) had been developed as inactivated pathogen products or toxoid vaccines. However, it was the coordination of immunization as a major public health tool from the 1950s onwards that led to the introduction of comprehensive vaccine programmes and their remarkable impact on child health that we enjoy today. In 1974, the World Health Organization launched the Expanded Programme on Immunization and a goal was set in 1977 to reach every child in the world with vaccines for diphtheria, pertussis, tetanus, poliomyelitis, measles and tuberculosis by 1990. Unfortunately, that goal has still not been reached; although global coverage of 3 doses of the diphtheria–tetanus–pertussis vaccine has risen to more than 85%, there are still more than 19 million children who did not receive basic vaccinations in 2019 (ref. 105 ).
What is in a vaccine?
A vaccine is a biological product that can be used to safely induce an immune response that confers protection against infection and/or disease on subsequent exposure to a pathogen. To achieve this, the vaccine must contain antigens that are either derived from the pathogen or produced synthetically to represent components of the pathogen. The essential component of most vaccines is one or more protein antigens that induce immune responses that provide protection. However, polysaccharide antigens can also induce protective immune responses and are the basis of vaccines that have been developed to prevent several bacterial infections, such as pneumonia and meningitis caused by Streptococcus pneumoniae , since the late 1980s 9 . Protection conferred by a vaccine is measured in clinical trials that relate immune responses to the vaccine antigen to clinical end points (such as prevention of infection, a reduction in disease severity or a decreased rate of hospitalization). Finding an immune response that correlates with protection can accelerate the development of and access to new vaccines 10 (Box 2 ).
Vaccines are generally classified as live or non-live (sometimes loosely referred to as ‘inactivated’) to distinguish those vaccines that contain attenuated replicating strains of the relevant pathogenic organism from those that contain only components of a pathogen or killed whole organisms (Fig. 2 ). In addition to the ‘traditional’ live and non-live vaccines, several other platforms have been developed over the past few decades, including viral vectors, nucleic acid-based RNA and DNA vaccines, and virus-like particles (discussed in more detail later).
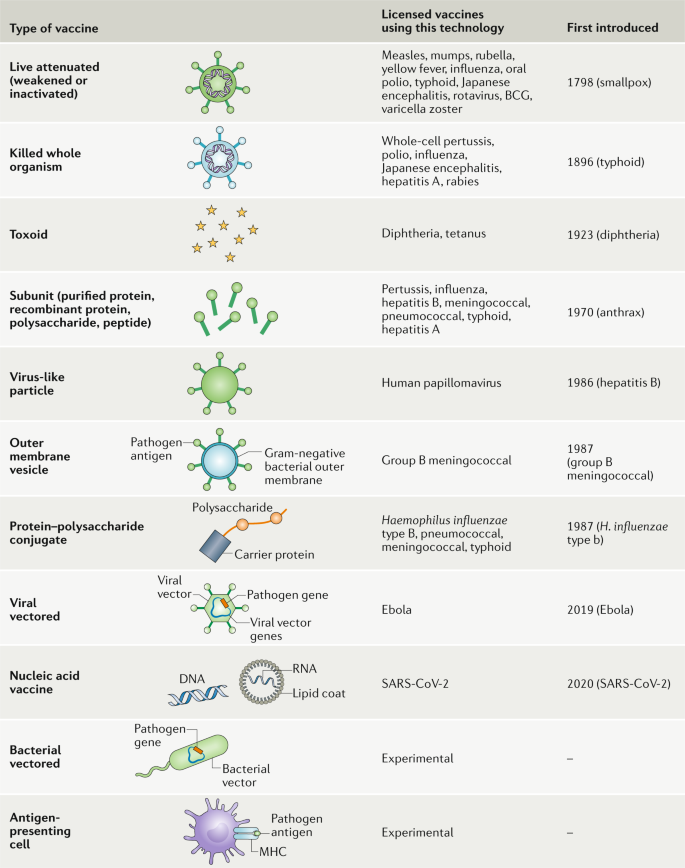
Schematic representation of different types of vaccine against pathogens; the text indicates against which pathogens certain vaccines are licensed and when each type of vaccine was first introduced. BCG, Mycobacterium bovis bacillus Calmette–Guérin.
The distinction between live and non-live vaccines is important. The former may have the potential to replicate in an uncontrolled manner in immunocompromised individuals (for example, children with some primary immunodeficiencies, or individuals with HIV infection or those receiving immunosuppressive drugs), leading to some restrictions to their use 11 . By contrast, non-live vaccines pose no risk to immunocompromised individuals (although they may not confer protection in those with B cell or combined immunodeficiency, as explained in more detail later).
Live vaccines are developed so that, in an immunocompetent host, they replicate sufficiently to produce a strong immune response, but not so much as to cause significant disease manifestations (for example, the vaccines for measles, mumps, rubella and rotavirus, oral polio vaccine, the Mycobacterium bovis bacillus Calmette–Guérin (BCG) vaccine for TB and live attenuated influenza vaccine). There is a trade-off between enough replication of the vaccine pathogen to induce a strong immune response and sufficient attenuation of the pathogen to avoid symptomatic disease. For this reason, some safe, live attenuated vaccines require multiple doses and induce relatively short-lived immunity (for example, the live attenuated typhoid vaccine, Ty21a) 12 , and other live attenuated vaccines may induce some mild disease (for example, about 5% of children will develop a rash and up to 15% fever after measles vaccination) 13 .
The antigenic component of non-live vaccines can be killed whole organisms (for example, whole-cell pertussis vaccine and inactivated polio vaccine), purified proteins from the organism (for example, acellular pertussis vaccine), recombinant proteins (for example, hepatitis B virus (HBV) vaccine) or polysaccharides (for example, the pneumococcal vaccine against S. pneumoniae ) (Fig. 2 ). Toxoid vaccines (for example, for tetanus and diphtheria) are formaldehyde-inactivated protein toxins that have been purified from the pathogen.
Non-live vaccines are often combined with an adjuvant to improve their ability to induce an immune response (immunogenicity). There are only a few adjuvants that are used routinely in licensed vaccines. However, the portfolio of adjuvants is steadily expanding, with liposome-based adjuvants and oil-in-water emulsions being licensed in the past few decades 14 . The mechanism of action of aluminium salts (alum), although extensively used as an adjuvant for more than 80 years, remains incompletely understood 15 , but there is increasing evidence that immune responses and protection can be enhanced by the addition of newer adjuvants that provide danger signals to the innate immune system . Examples of these novel adjuvants are the oil-in-water emulsion MF59, which is used in some influenza vaccines 16 ; AS01 , which is used in one of the shingles vaccines and the licensed malaria vaccine 17 ; and AS04 , which is used in a vaccine against human papillomavirus (HPV) 18 .
Vaccines contain other components that function as preservatives, emulsifiers (such as polysorbate 80) or stabilizers (for example, gelatine or sorbitol). Various products used in the manufacture of vaccines could theoretically also be carried over to the final product and are included as potential trace components of a vaccine, including antibiotics, egg or yeast proteins, latex, formaldehyde and/or gluteraldehyde and acidity regulators (such as potassium or sodium salts). Except in the case of allergy to any of these components, there is no evidence of risk to human health from these trace components of some vaccines 19 , 20 .
Box 2 Correlates of protection
The identification of correlates of protection is helpful in vaccine development as they can be used to compare products and to predict whether the use of an efficacious vaccine in a new population (for example, a different age group, medical background or geographical location) is likely to provide the same protection as that observed in the original setting. There is considerable confusion in the literature about the definition of a correlate of protection. For the purposes of this discussion, it is useful to separate out two distinct meanings. A mechanistic correlate of protection is the specific functional immune mechanism that is believed to confer protection. For example, antitoxin antibodies, which are induced by the tetanus toxoid vaccine, confer protection directly by neutralizing the activity of the toxin. A non-mechanistic correlate of protection does not in itself provide the protective function but has a statistical relationship with the mechanism of protection. An example of a non-mechanistic correlate of protection is total IgG antibody levels against pneumococci. These IgG antibodies contain the mechanistic correlate (thought to be a subset of opsonophagocytic antibodies ) but the mechanism of protection is not being directly measured. Correlates of protection can be measured in clinical trials if there are post-vaccination sera available from individuals who do or do not develop disease, although large-scale serum collection from participants is rarely undertaken in phase III clinical efficacy trials. An alternative approach is to estimate the correlates of protection by extrapolating from sero-epidemiological studies in a vaccinated population and relating the data to disease incidence in the population. Human challenge studies have also been used to determine correlates of protection, although the dose of challenge bacterium or virus and the experimental conditions may not relate closely to natural infection, which can limit the utility of these observations.
Vaccines induce antibodies
The adaptive immune response is mediated by B cells that produce antibodies (humoral immunity) and by T cells (cellular immunity). All vaccines in routine use, except BCG (which is believed to induce T cell responses that prevent severe disease and innate immune responses that may inhibit infection; see later), are thought to mainly confer protection through the induction of antibodies (Fig. 3 ). There is considerable supportive evidence that various types of functional antibody are important in vaccine-induced protection, and this evidence comes from three main sources: immunodeficiency states, studies of passive protection and immunological data.
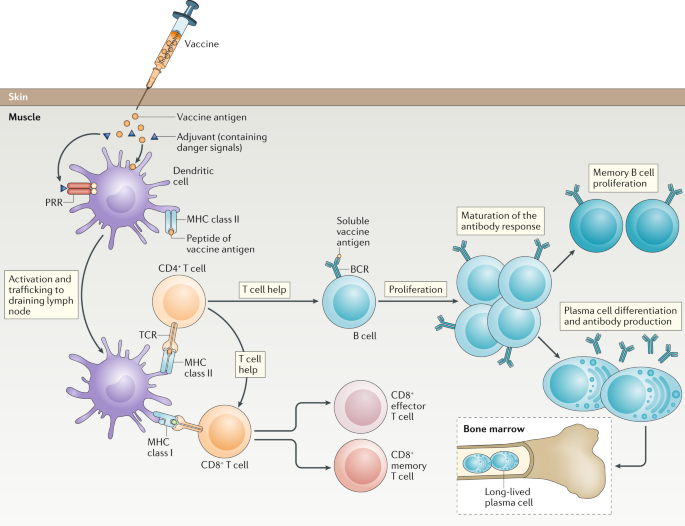
The immune response following immunization with a conventional protein antigen. The vaccine is injected into muscle and the protein antigen is taken up by dendritic cells, which are activated through pattern recognition receptors (PRRs) by danger signals in the adjuvant, and then trafficked to the draining lymph node. Here, the presentation of peptides of the vaccine protein antigen by MHC molecules on the dendritic cell activates T cells through their T cell receptor (TCR). In combination with signalling (by soluble antigen) through the B cell receptor (BCR), the T cells drive B cell development in the lymph node. Here, the T cell-dependent B cell development results in maturation of the antibody response to increase antibody affinity and induce different antibody isotypes. The production of short-lived plasma cells, which actively secrete antibodies specific for the vaccine protein, produces a rapid rise in serum antibody levels over the next 2 weeks. Memory B cells are also produced, which mediate immune memory. Long-lived plasma cells that can continue to produce antibodies for decades travel to reside in bone marrow niches. CD8 + memory T cells can proliferate rapidly when they encounter a pathogen, and CD8 + effector T cells are important for the elimination of infected cells.
Immunodeficiency states
Individuals with some known immunological defects in antibodies or associated immune components are particularly susceptible to infection with certain pathogens, which can provide insight into the characteristics of the antibodies that are required for protection from that particular pathogen. For example, individuals with deficiencies in the complement system are particularly susceptible to meningococcal disease caused by infection with Neisseria meningitidis 21 because control of this infection depends on complement-mediated killing of bacteria, whereby complement is directed to the bacterial surface by IgG antibodies. Pneumococcal disease is particularly common in individuals with reduced splenic function 22 (which may be congenital, resulting from trauma or associated with conditions such as sickle cell disease); S. pneumoniae bacteria that have been opsonized with antibody and complement are normally removed from the blood by phagocytes in the spleen, which are no longer present in individuals with hyposplenism. Antibody-deficient individuals are susceptible to varicella zoster virus (which causes chickenpox) and other viral infections, but, once infected, they can control the disease in the same way as an immunocompetent individual, so long as they have a normal T cell response 23 .
Passive protection
It has been clearly established that intramuscular or intravenous infusion of exogenous antibodies can provide protection against some infections. The most obvious example is that of passive transfer of maternal antibodies across the placenta, which provides newborn infants with protection against a wide variety of pathogens, at least for a few months after birth. Maternal vaccination with pertussis 24 , tetanus 25 and influenza 26 vaccines harnesses this important protective adaptation to reduce the risk of disease soon after birth and clearly demonstrates the role of antibodies in protection against these diseases. Vaccination of pregnant women against group B streptococci 27 and respiratory syncytial virus (RSV) 28 has not yet been shown to be effective at preventing neonatal or infant infection, but it has the potential to reduce the burden of disease in the youngest infants. Other examples include the use of specific neutralizing antibodies purified from immune donors to prevent the transmission of various viruses, including varicella zoster virus, HBV and measles virus 29 . Individuals with inherited antibody deficiency are without defence against serious viral and bacterial infections, but regular administration of serum antibodies from an immunocompetent donor can provide almost entirely normal immune protection for the antibody-deficient individual.
Immunological data
Increasing knowledge of immunology provides insights into the mechanisms of protection mediated by vaccines. For example, polysaccharide vaccines, which are made from the surface polysaccharides of invasive bacteria such as meningococci ( N. meningitidis ) 30 and pneumococci ( S. pneumoniae ) 31 , provide considerable protection against these diseases. It is now known that these vaccines do not induce T cell responses, as polysaccharides are T cell-independent antigens , and thus they must mediate their protection through antibody-dependent mechanisms. Protein–polysaccharide conjugate vaccines contain the same polysaccharides from the bacterial surface, but in this case they are chemically conjugated to a protein carrier (mostly tetanus toxoid, or diphtheria toxoid or a mutant protein derived from it, known as CRM 197 ) 32 , 33 , 34 . The T cells induced by the vaccine recognize the protein carrier (a T cell-dependent antigen ) and these T cells provide help to the B cells that recognize the polysaccharide, but no T cells are induced that recognize the polysaccharide and, thus, only antibody is involved in the excellent protection induced by these vaccines 35 . Furthermore, human challenge studies offer the opportunity to efficiently assess correlates of protection (Box 2 ) under controlled circumstances 36 , and they have been used to demonstrate the role of antibodies in protection against malaria 37 and typhoid 38 .
Vaccines need T cell help
Although most of the evidence points to antibodies being the key mediators of sterilizing immunity induced by vaccination, most vaccines also induce T cell responses. The role of T cells in protection is poorly characterized, except for their role in providing help for B cell development and antibody production in lymph nodes. From studies of individuals with inherited or acquired immunodeficiency, it is clear that whereas antibody deficiency increases susceptibility to acquisition of infection, T cell deficiency results in failure to control a pathogen after infection. For example, T cell deficiency results in uncontrolled and fatal varicella zoster virus infection, whereas individuals with antibody deficiency readily develop infection but recover in the same way as immunocompetent individuals. The relative suppression of T cell responses that occurs at the end of pregnancy increases the severity of infection with influenza and varicella zoster viruses 39 .
Although evidence for the involvement of T cells in vaccine-induced protection is limited, this is likely owing, in part, to difficulties in accessing T cells to study as only the blood is easily accessible, whereas many T cells are resident in tissues such as lymph nodes. Furthermore, we do not yet fully understand which types of T cell should be measured. Traditionally, T cells have been categorized as either cytotoxic (killer) T cells or helper T cells. Subtypes of T helper cells (T H cells) can be distinguished by their profiles of cytokine production. T helper 1 (T H 1) cells and T H 2 cells are mainly important for establishing cellular immunity and humoral immunity, respectively, although T H 1 cells are also associated with generation of the IgG antibody subclasses IgG1 and IgG3. Other T H cell subtypes include T H 17 cells (which are important for immunity at mucosal surfaces such as the gut and lung) and T follicular helper cells (located in secondary lymphoid organs, which are important for the generation of high-affinity antibodies (Fig. 3 )). Studies show that sterilizing immunity against carriage of S. pneumoniae in mice can be achieved by the transfer of T cells from donor mice exposed to S. pneumoniae 40 , which indicates that further investigation of T cell-mediated immunity is warranted to better understand the nature of T cell responses that could be harnessed to improve protective immunity.
Although somewhat simplistic, the evidence therefore indicates that antibodies have the major role in prevention of infection (supported by T H cells), whereas cytotoxic T cells are required to control and clear established infection.
Features of vaccine-induced protection
Vaccines have been developed over the past two centuries to provide direct protection of the immunized individual through the B cell-dependent and T cell-dependent mechanisms described above. As our immunological understanding of vaccines has developed, it has become apparent that this protection is largely manifested through the production of antibody. Another important feature of vaccine-induced protection is the induction of immune memory . Vaccines are usually developed to prevent clinical manifestations of infection. However, some vaccines, in addition to preventing the disease, may also protect against asymptomatic infection or colonization, thereby reducing the acquisition of a pathogen and thus its onward transmission, establishing herd immunity. Indeed, the induction of herd immunity is perhaps the most important characteristic of immunization programmes, with each dose of vaccine protecting many more individuals than the vaccine recipient. Some vaccines may also drive changes in responsiveness to future infections with different pathogens, so called non-specific effects, perhaps by stimulating prolonged changes in the activation state of the innate immune system.
Immune memory
In encountering a pathogen, the immune system of an individual who has been vaccinated against that specific pathogen is able to more rapidly and more robustly mount a protective immune response. Immune memory has been shown to be sufficient for protection against pathogens when the incubation period is long enough for a new immune response to develop (Fig. 4a ). For example, in the case of HBV, which has an incubation period of 6 weeks to 6 months, a vaccinated individual is usually protected following vaccination even if exposure to the virus occurs some time after vaccination and the levels of vaccine-induced antibody have already waned 41 . Conversely, it is thought that immune memory may not be sufficient for protection against rapidly invasive bacterial infections that can cause severe disease within hours or days following acquisition of the pathogen 42 (Fig. 4b ). For example, there is evidence in the case of both Haemophilus influenzae type B (Hib) and capsular group C meningococcal infection that individuals with vaccine-induced immune memory can still develop disease once their antibody levels have waned, despite mounting robust, although not rapid enough, memory responses 43 , 44 . The waning of antibody levels varies depending on the age of the vaccine recipient (being very rapid in infants as a result of the lack of bone marrow niches for B cell survival), the nature of the antigen and the number of booster doses administered. For example, the virus-like particles used in the HPV vaccine induce antibody responses that can persist for decades, whereas relatively short-term antibody responses are induced by pertussis vaccines; and the inactivated measles vaccine induces shorter-lived antibody responses than the live attenuated measles vaccine.
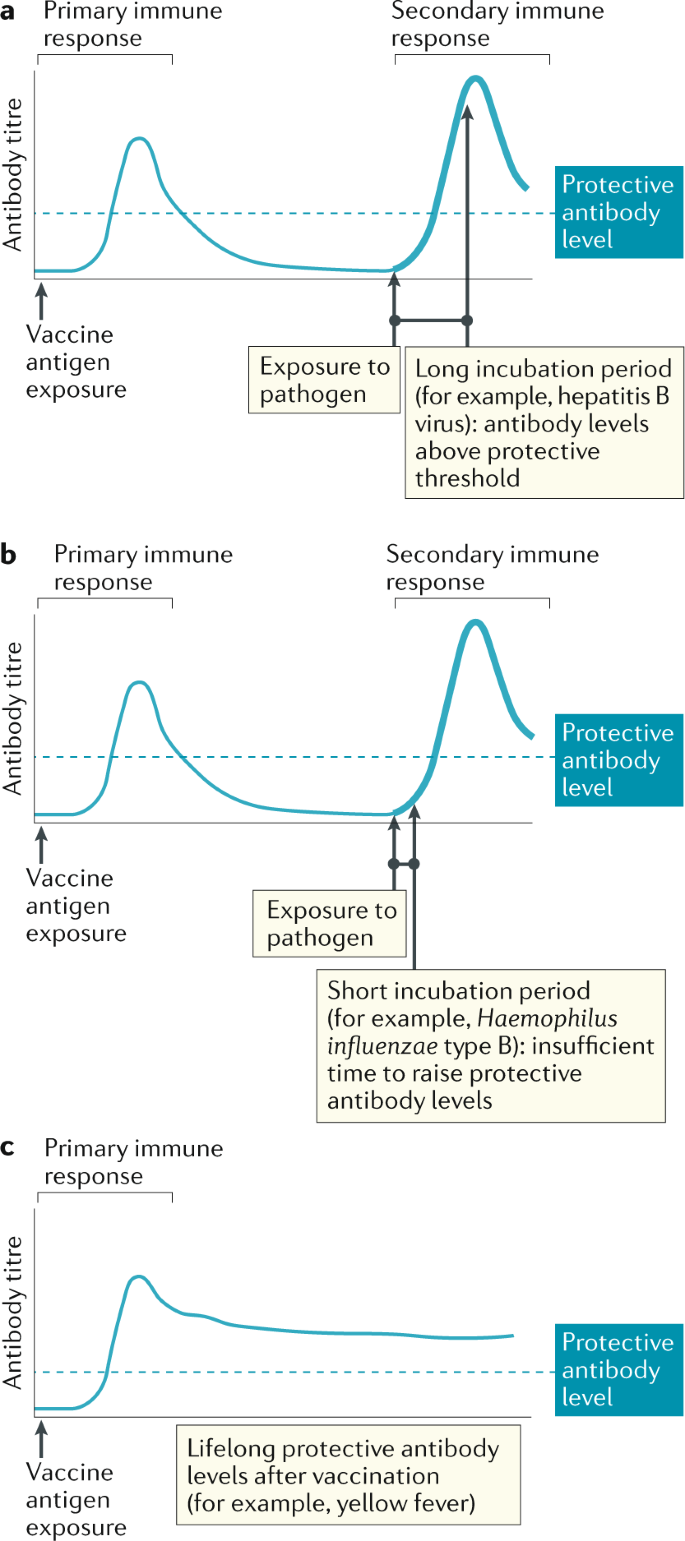
Antibody levels in the circulation wane after primary vaccination, often to a level below that required for protection. Whether immune memory can protect against a future pathogen encounter depends on the incubation time of the infection, the quality of the memory response and the level of antibodies induced by memory B cells. a | The memory response may be sufficient to protect against disease if there is a long incubation period between pathogen exposure and the onset of symptoms to allow for the 3–4 days required for memory B cells to generate antibody titres above the protective threshold. b | The memory response may not be sufficient to protect against disease if the pathogen has a short incubation period and there is rapid onset of symptoms before antibody levels have reached the protective threshold. c | In some cases, antibody levels after primary vaccination remain above the protective threshold and can provide lifelong immunity.
So, for infections that are manifest soon after acquisition of the pathogen, the memory response may be insufficient to control these infections and sustained immunity for individual protection through vaccination can be difficult to achieve. One solution to this is the provision of booster doses of vaccine through childhood (as is the case, for example, for diphtheria, tetanus, pertussis and polio vaccines), in an attempt to sustain antibody levels above the protective threshold. It is known that provision of five or six doses of tetanus 45 or diphtheria 46 vaccine in childhood provides lifelong protection, and so booster doses of these vaccines throughout adult life are not routine in most countries that can achieve high coverage with multiple childhood doses. Given that, for some infections, the main burden is in young children, continued boosting after the second year of life is not undertaken (for example, the invasive bacterial infections including Hib and capsular group B meningococci).
The exception is the pertussis vaccine, where the focus of vaccine programmes is the prevention of disease in infancy; this is achieved both by direct vaccination of infants as well as by the vaccination of other age groups, including adolescents and pregnant women in some programmes, to reduce transmission to infants and provide protection by antibody transfer across the placenta. Notably, in high-income settings, many countries (starting in the 1990s) have switched to using the acellular pertussis vaccine, which is less reactogenic than (and therefore was thought to be preferable to) the older whole-cell pertussis vaccine that is still used in most low-income countries. It is now apparent that acellular pertussis vaccine induces a shorter duration of protection against clinical pertussis and may be less effective against bacterial transmission than is the whole-cell pertussis vaccine 47 . Many high-income countries have observed a rise in pertussis cases since the introduction of the acellular vaccine, a phenomenon that is not observed in low-income nations using the whole-cell vaccine 48 .
By contrast, lifelong protection seems to be the rule following a single dose with some of the live attenuated viral vaccines, such as yellow fever vaccine 49 (Fig. 4c ), although it is apparent that protection is incomplete with others. In the case of varicella zoster and measles–mumps vaccines, some breakthrough cases are described during disease outbreaks among those individuals who have previously been vaccinated, although it is unclear whether this represents a group in whom immunity has waned (and who therefore needed booster vaccination) or a group for whom the initial vaccine did not induce a successful immune response. Breakthrough cases are less likely in those individuals who have had two doses of measles–mumps–rubella vaccine 50 or varicella zoster vaccine 51 , and cases that do occur are usually mild, which indicates that there is some lasting immunity to the pathogen.
An illustration of the complexity of immune memory and the importance of understanding its underlying immunological mechanisms in order to improve vaccination strategies is provided by the concept of ‘original antigenic sin’. This phenomenon describes how the immune system fails to generate an immune response against a strain of a pathogen if the host was previously exposed to a closely related strain, and this has been demonstrated in several infections, including dengue 52 and influenza 53 . This might have important implications for vaccine development if only a single pathogen strain or pathogen antigen is included in a vaccine, as vaccine recipients might then have impaired immune responses if later exposed to different strains of the same pathogen, potentially putting them at increased risk of infection or more severe disease. Strategies to overcome this include the use of adjuvants that stimulate innate immune responses, which can induce sufficiently cross-reactive B cells and T cells that recognize different strains of the same pathogen, or the inclusion of as many strains in a vaccine as possible, the latter approach obviously being limited by the potential of new strains to emerge in the future 54 .
Herd immunity
Although direct protection of individuals through vaccination has been the focus of most vaccine development and is crucial to demonstrate for the licensure of new vaccines, it has become apparent that a key additional component of vaccine-induced protection is herd immunity, or more correctly ‘herd protection’ (Fig. 5 ). Vaccines cannot protect every individual in a population directly, as some individuals are not vaccinated for various reasons and others do not mount an immune response despite vaccination. Fortunately, however, if enough individuals in a population are vaccinated, and if vaccination prevents not only the development of disease but also infection itself (discussed in more detail below), transmission of the pathogen can be interrupted and the incidence of disease can fall further than would be expected, as a result of the indirect protection of individuals who would otherwise be susceptible.
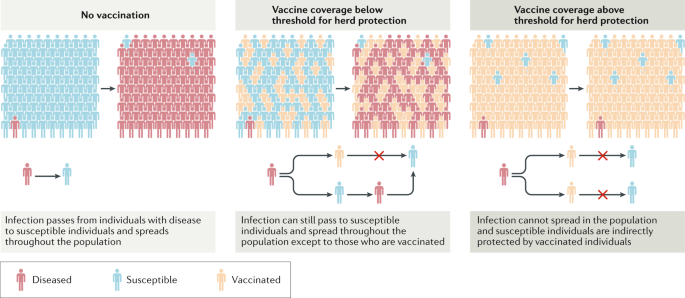
The concept of herd immunity for a highly contagious disease such as measles. Susceptible individuals include those who have not yet been immunized (for example, being too young), those who cannot be immunized (for example, as a result of immunodeficiency), those for whom the vaccine did not induce immunity, those for whom initial vaccine-induced immunity has waned and those who refused immunization.
For highly transmissible pathogens, such as those causing measles or pertussis, around 95% of the population must be vaccinated to prevent disease outbreaks, but for less transmissible organisms a lower percentage of vaccine coverage may be sufficient to have a substantial impact on disease (for example, for polio, rubella, mumps or diphtheria, vaccine coverage can be ≤86%). For influenza, the threshold for herd immunity is highly variable from season to season and is also confounded by the variability in vaccine effectiveness each year 55 . Modest vaccine coverage, of 30–40%, is likely to have an impact on seasonal influenza epidemics, but ≥80% coverage is likely to be optimal 56 . Interestingly, there might be a downside to very high rates of vaccination, as the absence of pathogen transmission in that case will prevent natural boosting of vaccinated individuals and could lead to waning immunity if booster doses of vaccine are not used.
Apart from tetanus vaccine, all other vaccines in the routine immunization schedule induce some degree of herd immunity (Fig. 5 ), which substantially enhances population protection beyond that which could be achieved by vaccination of the individual only. Tetanus is a toxin-mediated disease acquired through infection of breaks in the skin contaminated with the toxin-producing bacteria Clostridium tetani from the environment — so, vaccination of the community with the tetanus toxoid will not prevent an unvaccinated individual acquiring the infection if they are exposed. As an example of the success of herd immunity, vaccination of children and young adults (up to 19 years of age) with capsular group C meningococcal vaccine in a mass campaign in 1999 resulted in almost complete elimination of disease from the UK in adults as well as children 57 . Currently, the strategy for control of capsular groups A, C, W and Y meningococci in the UK is vaccination of adolescents, as they are mainly responsible for transmission and vaccine-mediated protection of this age group leads to community protection through herd immunity 58 . The HPV vaccine was originally introduced to control HPV-induced cervical cancer, with vaccination programmes directed exclusively at girls, but it was subsequently found to also provide protection against HPV infection in heterosexual boys through herd immunity, which led to a marked reduction in the total HPV burden in the population 59 , 60 .
Prevention of infection versus disease
Whether vaccines prevent infection or, rather, the development of disease after infection with a pathogen is often difficult to establish, but improved understanding of this distinction could have important implications for vaccine design. BCG vaccination can be used as an example to illustrate this point, as there is some evidence for the prevention of both disease and infection. BCG vaccination prevents severe disease manifestations such as tuberculous meningitis and miliary TB in children 61 and animal studies have shown that BCG vaccination reduces the spread of M. tuberculosis bacteria in the blood, mediated by T cell immunity 62 , thereby clearly showing that vaccination has protective effects against the development of disease after infection. However, there is also good evidence that BCG vaccination reduces the risk of infection. In a TB outbreak at a school in the UK, 29% of previously BCG-vaccinated children had a memory T cell response to infection, as indicated by a positive interferon-γ release assay , as compared with 47% of the unvaccinated children 63 . A similar effect was seen when studying Indonesian household members of patients with TB, who had a 45% reduced chance of developing a positive interferon-γ release assay response to M. tuberculosis if they had previously been BCG vaccinated 64 . The lack of a T cell response in previously vaccinated individuals indicates that the BCG vaccine induces an innate immune response that results in ‘early clearance’ of the bacteria and prevents infection that induces an adaptive immune response. It will be hugely valuable for future vaccine development to better understand the induction of such protective innate immune responses so that they might be reproduced for other pathogens.
In the case of the current pandemic of the virus SARS-CoV-2, a vaccine that prevents severe disease and disease-driven hospitalization could have a substantial public health impact. However, a vaccine that could also block acquisition of the virus, and thus prevent both asymptomatic and mild infection, would have much larger impact by reducing transmission in the community and potentially establishing herd immunity.
Non-specific effects
Several lines of evidence indicate that immunization with some vaccines perturbs the immune system in such a way that there are general changes in immune responsiveness that can increase protection against unrelated pathogens 65 . This phenomenon has been best described in humans in relation to BCG and measles vaccines, with several studies showing marked reductions in all-cause mortality when these vaccines are administered to young children that are far beyond the expected impact from the reduction in deaths attributed to TB or measles, respectively 66 . These non-specific effects may be particularly important in high-mortality settings, but not all studies have identified the phenomenon. Although several immunological mechanisms have been proposed, the most plausible of which is that epigenetic changes can occur in innate immune cells as a result of vaccination, there are no definitive studies in humans that link immunological changes after immunization with important clinical end points, and it remains unclear how current immunization schedules might be adapted to improve population protection through non-specific effects. Of great interest in the debate, recent studies have indicated that measles disease casts a prolonged ‘shadow’ over the immune system, with depletion of existing immune memory, such that children who have had the disease have an increased risk of death from other causes over the next few years 67 , 68 . In this situation, measles vaccination reduces mortality from measles as well as the unconnected diseases that would have occurred during the ‘shadow’, resulting in a benefit that seems to be non-specific but actually relates directly to the prevention of measles disease and its consequences. This illustrates a limitation of vaccine study protocols: as these are usually designed to find pathogen-specific effects, the possibility of important non-specific effects cannot be assessed.
Factors affecting vaccine protection
The level of protection afforded by vaccination is affected by many genetic and environmental factors, including age, maternal antibody levels, prior antigen exposure, vaccine schedule and vaccine dose. Although most of these factors cannot be readily modified, age of vaccination and schedule of vaccination are important and key factors in planning immunization programmes. The vaccine dose is established during early clinical development, based on optimal safety and immunogenicity. However, for some populations, such as older adults, a higher dose might be beneficial, as has been shown for the influenza vaccine 69 , 70 . Moreover, intradermal vaccination has been shown to be immunogenic at much lower (fractional) doses than intramuscular vaccination for influenza, rabies and HBV vaccines 71 .
Age of vaccination
The highest burden of and mortality from infectious disease occur in the first 5 years of life, with the youngest infants being most affected. For this reason, immunization programmes have largely focused on this age group where there is the greatest benefit from vaccine-induced protection. Although this makes sense from an epidemiological perspective, it is somewhat inconvenient from an immunological perspective as the induction of strong immune responses in the first year of life is challenging. Indeed, vaccination of older children and adults would induce stronger immune responses, but would be of little value if those who would have benefited from vaccination have already succumbed to the disease.
It is not fully understood why immune responses to vaccines are not as robust in early infancy as they are in older children. One factor, which is increasingly well documented, is interference from maternal antibody 72 — acquired in utero through the placenta — which might reduce antigen availability, reduce viral replication (in the case of live viral vaccines such as measles 73 ) or perhaps regulate B cell responses. However, there is also evidence that there is a physiological age-dependent increase in antibody responses in infancy 72 . Furthermore, bone marrow niches to support B cells are limited in infancy, which might explain the very short-lived immune responses that are documented in the first year of life 74 . For example, after immunization with 2 doses of the capsular group C meningococcal vaccine in infancy, only 41% of infants still had protective levels of antibody by the time of the booster dose, administered 7 months later 75 .
In the case of T cell-independent antigens — in other words, plain polysaccharides from Hib, typhoid-causing bacteria, meningococci and pneumococci — animal data indicate that antibody responses depend on development of the marginal zone of the spleen, which is required for the maturation of marginal zone B cells, and this does not occur until around 18 months of age in human infants 76 . These plain polysaccharide vaccines do not induce memory B cells (Fig. 6 ) and, even in adults, provide protection for just 2–3 years, with protection resulting from antibody produced by plasma cells derived from marginal zone B cells 77 . However, converting plain polysaccharide vaccines into T cell-dependent protein–polysaccharide conjugate vaccines, which are immunogenic from 2 months of age and induce immune memory, has transformed prevention of disease caused by the encapsulated bacteria (pneumococci, Hib and meningococci) over the past three decades 78 . These are the most important invasive bacterial pathogens of childhood, causing most cases of childhood meningitis and bacterial pneumonia, and the development of the conjugate vaccine technology in the 1980s has transformed global child health 9 .
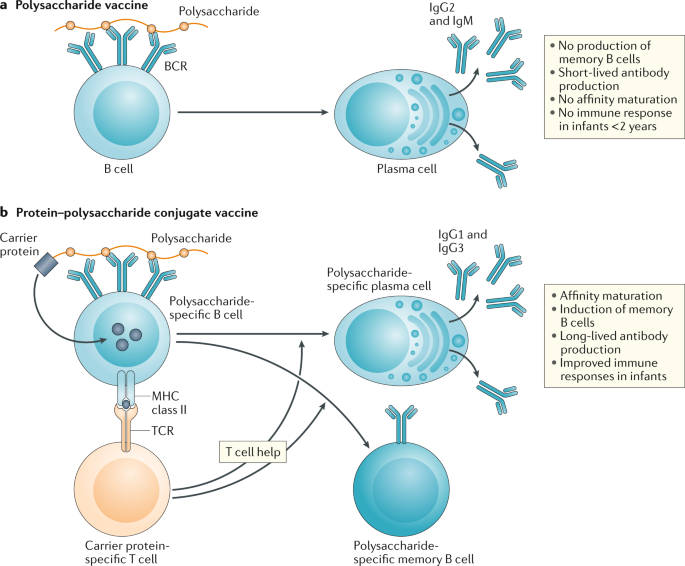
a | Polysaccharide vaccines induce antibody-producing plasma cells by cross-linking the B cell receptor (BCR). However, affinity maturation of the antibody response and the induction of memory B cells do not occur. b | Protein–polysaccharide conjugate vaccines can engage T cells that recognize the carrier protein, as well as B cells that recognize the polysaccharide. T cells provide help to B cells, leading to affinity maturation and the production of both plasma cells and memory B cells. TCR, T cell receptor. Adapted from ref. 35 , Springer Nature Limited.
Immune responses are also poor in the older population and most of the vaccines used in older adults offer limited protection or a limited duration of protection, particularly among those older than 75 years of age. The decline in immune function with age (known as immunosenescence) has been well documented 79 but, despite the burden of infection in this age group and the increasing size of the population, has not received sufficient attention so far amongst immunologists and vaccinologists. Interestingly, some have raised the hypothesis that chronic infection with cytomegalovirus (CMV) might have a role in immunosenescence through unfavourable effects on the immune system, including clonal expansion of CMV-specific T cell populations, known as ‘memory inflation’, and reduced diversity of naive T cells 80 , 81 .
In high-income countries, many older adults receive influenza, pneumococcal and varicella zoster vaccines, although data showing substantial benefits of these vaccines in past few decades in the oldest adults (more than 75 years of age) are lacking. However, emerging data following the recent development and deployment of new-generation, high-dose or adjuvanted influenza vaccines 82 and an adjuvanted glycoprotein varicella zoster vaccine 83 suggest that the provision of additional signals to the immune system by certain adjuvants (such as AS01 and MF59) can overcome immunosenescence. It is now necessary to understand how and why, and to use this knowledge to expand options for vaccine-induced protection at the extremes of life.
Schedule of vaccination
For most vaccines that are used in the first year of life, 3–4 doses are administered by 12 months of age. Conventionally, in human vaccinology, ‘priming’ doses are all those administered at less than 6 months of age and the ‘booster’ dose is given at 9–12 months of age. So, for example, the standard WHO schedule for diphtheria–tetanus–pertussis-containing vaccines (which was introduced in 1974 as part of the Expanded Programme on Immunization 84 ) consists of 3 priming doses at 6, 10 and 14 weeks of age with no booster. This schedule was selected to provide early protection before levels of maternal antibody had waned (maternal antibody has a half-life of around 30–40 days 85 , so very little protection is afforded to infants from the mother beyond 8–12 weeks of age) and because it was known that vaccine compliance is better when doses are given close together. However, infant immunization schedules around the world are highly variable — few high-income or middle-income countries use the Expanded Programme on Immunization schedule — and were largely introduced with little consideration of how best to optimize immune responses. Indeed, schedules that start later at 8–12 weeks of age (when there is less interference from maternal antibody) and have longer gaps between doses (8 weeks rather than 4 weeks) are more immunogenic. A large number of new vaccines have been introduced since 1974 as a result of remarkable developments in technology, but these have generally been fitted into existing schedules without taking into account the optimal scheduling for these new products. The main schedules used globally for diphtheria–tetanus–pertussis vaccine are presented in Supplementary Table 1 , and the changes to the UK immunization schedule since 1963 are presented in Supplementary Table 2 . It should also be noted that surveys show vaccines are rarely delivered on schedule in many countries and, thus, the published schedule may not be how vaccines are actually delivered on the ground. This is particularly the case in remote areas (for example, where health professionals only visit occasionally) and regions with limited or chaotic health systems, leaving children vulnerable to infection.
Safety and side effects of vaccines
Despite the public impression that vaccines are associated with specific safety concerns, the existing data indicate that vaccines are remarkably safe as interventions to defend human health. Common side effects, particularly those associated with the early innate immune response to vaccines, are carefully documented in clinical trials. Although rare side effects might not be identified in clinical trials, vaccine development is tightly controlled and robust post-marketing surveillance systems are in place in many countries, which aim to pick these up if they do occur. This can make the process of vaccine development rather laborious but is appropriate because, unlike most drugs, vaccines are used for prophylaxis in a healthy population and not to treat disease. Perhaps because vaccines work so well and the diseases that they prevent are no longer common, there have been several spurious associations made between vaccines and various unrelated health conditions that occur naturally in the population. Disentangling incorrect claims of vaccine harm from true vaccine-related adverse events requires very careful epidemiological studies.
Common side effects
Licensure of a new vaccine normally requires safety studies involving from 3,000 to tens of thousands of individuals. Thus, common side effects are very well known and are published by the regulator at the time of licensure. Common side effects of many vaccines include injection site pain, redness and swelling and some systemic symptoms such as fever, malaise and headache. All of these side effects, which occur in the first 1–2 days following vaccination, reflect the inflammatory and immune responses that lead to the successful development of vaccine-induced protection. About 6 days after measles–mumps–rubella vaccination, about 10% of 12-month-old infants develop a mild viraemia, which can result in fever and rash, and occasionally febrile convulsions (1 in 3,000) 86 . Although these side effects are self-limiting and relatively mild — and are trivial in comparison with the high morbidity and mortality of the diseases from which the vaccines protect — they can be very worrying for parents and their importance is often underestimated by clinicians who are counselling families about immunization.
Immunodeficiency and vaccination
Most vaccines in current use are inactivated, purified or killed organisms or protein and/or polysaccharide components of a pathogen; as they cannot replicate in the vaccine recipient, they are thus not capable of causing any significant side effects, resulting in very few contraindications for their use. Even in immunocompromised individuals, there is no risk from use of these vaccines, although the induction of immunity may not be possible, depending on the nature of the immune system defect. More caution is required for the use of live attenuated, replicating vaccines (such as yellow fever, varicella zoster, BCG and measles vaccines) in the context of individuals with T cell immunodeficiency as there is a theoretical risk of uncontrolled replication, and live vaccines are generally avoided in this situation 87 . A particular risk of note is from the yellow fever vaccine, which is contraindicated in individuals with T cell immunodeficiency and occasionally causes a severe viscerotropic or neurotropic disease in individuals with thymus disease or after thymectomy, in young infants and adults more than 60 years of age 88 . In individuals with antibody deficiency, there may be some merit in the use of routine live vaccines, as T cell memory may be induced that, although unlikely to prevent future infection, could improve control of the disease if infection occurs.
The myth of antigenic overload
An important parental concern is that vaccines might overwhelm their children’s immune systems. In a telephone survey in the USA, 23% of parents agreed with the statement ‘Children get more immunizations than are good for them’, and 25% indicated that they were concerned that their child’s immune system could be weakened by too many immunizations 89 . However, there is ample evidence to disprove these beliefs. Although the number of vaccines in immunization programmes has increased, the total number of antigens has actually decreased from more than 3,200 to approximately 320 as a result of discontinuing the smallpox vaccine and replacing the whole-cell pertussis vaccine with the acellular vaccine 90 , 91 . Vaccines comprise only a small fraction of the antigens that children are exposed to throughout normal life, with rapid bacterial colonization of the gastrointestinal tract after birth, multiple viral infections and environmental antigens. Moreover, multiple studies have shown that children who received vaccinations had a similar, or even reduced, risk of unconnected infections in the following period 92 , 93 , 94 , 95 . Looking at children who presented to the emergency department with infections not included in the vaccine programme, there was no difference in terms of their previous antigen exposure by vaccination 96 .
Significant rare side effects
Serious side effects from vaccines are very rare, with anaphylaxis being the most common of these rare side effects for parenteral vaccines , occurring after fewer than one in a million doses 97 . Individuals with known allergies (such as egg or latex) should avoid vaccines that may have traces of these products left over from the production process with the specific allergen, although most cases of anaphylaxis are not predictable in advance but are readily managed if vaccines are administered by trained health-care staff.
Very rare side effects of vaccines are not usually observed during clinical development, with very few documented, and they are only recognized through careful surveillance in vaccinated populations. For example, there is a very low risk of idiopathic thrombocytopenic purpura (1 in 24,000 vaccine recipients) after measles vaccination 86 . From 1 in 55,000 to 1 in 16,000 recipients of an AS03-adjuvanted 2009 pandemic H1N1 influenza vaccine 98 , 99 , who had a particular genetic susceptibility (HLA DQB1*0602) 100 , developed narcolepsy , although the debate continues about whether the trigger was the vaccine, the adjuvant or some combination, perhaps with the circulating virus also having a role.
Despite widespread misleading reporting about links between the measles–mumps–rubella vaccine and autism from the end of the 1990s, there is no evidence that any vaccines or their components cause autism 101 , 102 . Indeed, the evidence now overwhelmingly shows that there is no increased risk of autism in vaccinated populations. Thiomersal (also known as thimerosal) is an ethyl mercury-containing preservative that has been used widely in vaccines since the 1930s without any evidence of adverse events associated with it, and there is also no scientific evidence of any link between thiomersal and autism despite spurious claims about this 102 . Thiomersal has been voluntarily withdrawn from most vaccines by manufacturers as a precautionary measure rather than because of any scientific evidence of lack of safety and is currently used mainly in the production of whole-cell pertussis vaccines.
The risk of hospitalization, death or long-term morbidity from the diseases for which vaccines have been developed is so high that the risks of common local and systemic side effects (such as sore arm and fever) and the rare more serious side effects are far outweighed by the massive reductions in disease achieved through vaccination. Continuing assessment of vaccine safety post licensure is important for the detection of rare and longer-term side effects, and efficient reporting systems need to be in place to facilitate this 103 . This is particularly important in a pandemic situation, such as the COVID-19 pandemic, as rapid clinical development of several vaccines is likely to take place and large numbers of people are likely to be vaccinated within a short time.
Challenges to vaccination success
Vaccines only work if they are used. Perhaps the biggest challenge to immunization programmes is ensuring that the strong headwinds against deployment, ranging from poor infrastructure and lack of funding to vaccine hesitancy and commercial priorities, do not prevent successful protection of the most vulnerable in society. It is noteworthy that these are not classical scientific challenges, although limited knowledge about which antigens are protective, which immune responses are needed for protection and how to enhance the right immune responses, particularly in the older population, are also important considerations.
Access to vaccines
The greatest challenge for protection of the human population against serious infectious disease through vaccination remains access to vaccines and the huge associated inequity in access. Access to vaccines is currently limited, to varying degrees in different regions, by the absence of a health infrastructure to deliver vaccines, the lack of convenient vaccine provision for families, the lack of financial resources to purchase available vaccines (at a national, local or individual level) and the marginalization of communities in need. This is perhaps the most pressing issue for public health, with global vaccine coverage having stalled; for example, coverage for diphtheria–tetanus–pertussis-containing vaccines has only risen from 84% to 86% since 2010 (ref. 104 ). However, this figure hides huge regional variation, with near 100% coverage in some areas and almost no vaccinated children in others. For the poorest countries in the world, Gavi, the Vaccine Alliance provides funding to assist with new vaccine introductions and has greatly accelerated the broadening of access to new vaccines that were previously only accessible to high-income countries. However, this still leaves major financial challenges for countries that do not meet the criteria to be eligible for Gavi funding but still cannot afford new vaccines. Inequity remains, with approximately 14 million children not receiving any vaccinations and another 5.7 million children being only partially vaccinated in 2019 (ref. 105 ).
Other important issues can compromise vaccine availability and access. For example, most vaccines must be refrigerated at 2–8 °C, requiring the infrastructure and capacity for cold storage and a cold chain to the clinic where the vaccine is delivered, which is limited in many low-income countries. The route of administration can also limit access; oral vaccines (such as rotavirus, polio or cholera vaccines) and nasal vaccines (such as live attenuated influenza vaccine) can be delivered rapidly on a huge scale by less-skilled workers, whereas most vaccines are injected, which requires more training to administer and takes longer. Nevertheless, these hurdles can be overcome: in Sindh Province, Pakistan, 10 million doses of injected typhoid conjugate vaccine were administered to children to control an outbreak of extensively drug-resistant typhoid in just a few weeks at the end of 2019 (ref. 106 ).
The anti-vaccination movement
Despite access being the main issue affecting global vaccine coverage, a considerable focus is currently on the challenges posed by the anti-vaccination movement, largely as a result of worrying trends of decreasing vaccine coverage in high-income settings, leading to outbreaks of life-threatening infectious diseases, such as measles. In 2018, there were 140,000 deaths from measles worldwide, and the number of cases in 2019 was the highest in any year since 2006 (ref. 107 ). Much has been written about the dangerous role of social media and online search engines in the spread of misinformation about vaccines and the rise of the anti-vaccination movement, but scientists are also at fault for failing to effectively communicate the benefits of vaccination to a lay public. If this is to change, scientists do not need to counter or engage with the anti-vaccination movement but to use their expertise and understanding to ensure effective communication about the science that underpins our remarkable ability to harness the power of the immune system through vaccination to defend the health of our children.
Commercial viability
A third important issue is the lack of vaccines for some diseases for which there is no commercial incentive for development. Typically, these are diseases that have a restricted geographical spread (such as Rift Valley fever, Ebola, Marburg disease or plague) or occur in sporadic outbreaks and only affect poor or displaced communities (such as Ebola and cholera). Lists of outbreak pathogens have been published by various agencies including the WHO 108 , and recent funding initiatives, including those from US and European governments, have increased investment in the development of orphan vaccines . The Coalition for Epidemic Preparedness Innovations (CEPI) is set to have a major role in funding and driving the development of vaccines against these pathogens.
Immunological challenges
For other pathogens, there is likely to be a commercial market but there are immunological challenges for the development of new vaccines. For example, highly variable pathogens, including some with a large global distribution such as HIV and hepatitis C virus, pose a particular challenge. The genetic diversity of these pathogens, which occurs both between and within hosts, makes it difficult to identify an antigen that can be used to immunize against infection. In the case of HIV, antibodies can be generated that neutralize the virus, but the rapid mutation of the viral genome means that the virus can evade these responses within the same host. Some individuals do produce broadly neutralizing antibodies naturally, which target more conserved regions of the virus, leading to viral control, but it is not clear how to robustly induce these antibodies with a vaccine. Indeed, several HIV vaccines have been tested in clinical trials that were able to induce antibody responses (for example, RV144 vaccine showed 31% protection 109 ) and/or T cell responses, but these vaccines have not shown consistent evidence of protection in follow-up studies, and several studies found an increased risk of infection among vaccine recipients 110 .
For other pathogens, such as Neisseria gonorrhoeae (which causes gonorrhoea) and Treponema pallidum (which causes syphilis), antigenic targets for protective immune responses have not yet been determined, partly owing to limited investment and a poor understanding of the mechanisms of immunity at mucosal surfaces, or have thus far only resulted in limited protection. For example, the licensed malaria vaccine, RTSS, provides only 30–40% protection and further work is needed to develop suitable products 111 . New malaria vaccines in development target more conserved antigens on the parasite surface or target different stages of the parasite life cycle. Combinations of these approaches in a vaccine (perhaps targeting multiple stages of the life cycle), together with anti-vector strategies such as the use of genetically modified mosquitoes or Wolbachia bacteria to infect mosquitoes and reduce their ability to carry mosquito parasites 112 , as well as mosquito-bite avoidance, have the potential to markedly reduce malaria parasite transmission.
Seasonal influenza vaccines have, in recent decades, been used to protect vulnerable individuals in high-income countries, including older adults, children and individuals with co-morbidities that increase risk of severe influenza. These vaccines are made from virus that is grown in eggs; purified antigen, split virions or whole virions can be included in the final vaccine product. The vaccines take around 6 months to manufacture and have highly variable efficacy from one season to another, partly owing to the difficulty in predicting which virus strain will be circulating in the next influenza season, so that the vaccine strain may not match the strain causing disease 113 . Another issue that is increasingly recognized is egg adaptation, whereby the vaccine strain of virus becomes adapted to the egg used for production, leading to key mutations that mean it is not well matched to, and does not protect against, the circulating viral strain 114 . Vaccine-induced protection might be improved by the development of mammalian or insect cell-culture systems for growing influenza virus to avoid egg adaptation, and the use of MF59-adjuvanted vaccines and high-dose influenza vaccines to improve immune responses. Because of the cost of purchasing seasonal influenza vaccines annually, and the problem of antigenic variability, the search for a universal influenza vaccine receives considerable attention, with a particular focus on vaccines that induce T H cells or antibodies to conserved epitopes 115 , but there are currently no products in late-stage development.
Although BCG is the most widely used vaccine globally, with 89% of the world population receiving it in 2018 (ref. 105 ), there is still a huge global burden of TB and it is clear that more effective TB vaccines are needed. However, the optimal characteristics of a prophylactic TB vaccine, which antigens should be included and the nature of protective immunity remain unknown, despite more than 100 years of TB vaccine research. A viral vector expressing a TB protein, 85A, has been tested in a large TB-prevention trial in South Africa but this vaccine did not show protection, which was attributed by the authors to poor immunogenicity in the vaccinated children 116 . However, the publication of a study in 2019 showing that a novel TB vaccine, M72/AS01E (an AS01-adjuvanted vaccine containing the M. tuberculosis antigens MTB32A and MTB39A), could limit progression to active TB disease in latently infected individuals with efficacy of 50% over 3 years gives a glimmer of hope that TB control may be realized in the future by novel vaccine approaches 117 . Questions remain about the duration of the effect, but the demonstrated efficacy can now be interrogated thoroughly to determine the nature of protective immunity against TB.
Future vaccine development
There are several important diseases for which new vaccines are needed to reduce morbidity and mortality globally, which are likely to have a market in both high-income and low-income countries, including vaccines for group B Streptococcus (a major cause of neonatal meningitis), RSV and CMV. Group B Streptococcus vaccines are currently in trials of maternal vaccination, with the aim of inducing maternal antibodies that cross the placenta and protect the newborn passively 118 . RSV causes a lower respiratory tract infection, bronchiolitis, in infancy and is the commonest cause of infant hospitalization in developed countries and globally one of the leading causes of death in those less than 12 months of age. As many as 60 new RSV vaccine candidates are in development as either maternal vaccines or infant vaccines, or involving immunization with RSV-specific monoclonal antibodies that have an extended half-life. A licensed RSV vaccine would have a huge impact on infant health and paediatric hospital admissions. CMV is a ubiquitous herpesvirus that is responsible for a significant burden of disease in infants; 15–20% of congenitally infected children develop long-term sequelae, most importantly sensorineural hearing loss, and CMV thus causes more congenital disease than any other single infectious agent. A vaccine that effectively prevents congenital infection would provide significant individual and public health benefits. A lack of understanding of the nature of protective immunity against CMV has hampered vaccine development in the past, but the pipeline is now more promising 119 , 120 .
Another major line of development of new vaccines is to combat hospital-acquired infections, particularly with antibiotic-resistant Gram-positive bacteria (such as Staphylococcus aureus ) that are associated with wound infections and intravenous catheters and various Gram-negative organisms (such as Klebsiella spp. and Pseudomonas aeruginosa ). Progress has been slow in this field and an important consideration will be targeting products to the at-risk patient groups before hospital admission or surgery.
Perhaps the largest area of growth for vaccine development is for older adults, with few products aimed specifically at this population currently. With the population of older adults set to increase substantially (the proportion of the population who are more than 60 years of age is expected to increase from 12% to 22% by 2050 (ref. 121 )), prevention of infection in this population should be a public health priority. Efforts to better understand immunosenescence and how to improve vaccine responses in the oldest adults are a major challenge for immunologists today.
Novel technologies
Important challenges to overcome in the following years are genetic diversity (for example, of viruses such as HIV, hepatitis C virus and influenza), the requirement for a broader immune response including T cells for protection against diseases such as TB and malaria, and the need to swiftly respond to emerging pathogens and outbreak situations. Traditionally, vaccine development takes more than 10 years 122 , but the COVID-19 pandemic has demonstrated the urgency for vaccine technologies that are flexible and facilitate rapid development, production and upscaling 123 .
Novel technologies to combat these hurdles will include platforms that allow for improved antigen delivery and ease and speed of production, application of structural biology and immunological knowledge to aid enhanced antigen design and discovery of better adjuvants to improve immunogenicity. Fortunately, recent advances in immunology, systems biology, genomics and bio-informatics offer great opportunities to improve our understanding of the induction of immune responses by vaccines and to transform vaccine development through increasingly rational design 124 .
New platforms include viral vectored vaccines and nucleic acid-based vaccines. Antigen-presenting cells such as dendritic cells, T cell-based vaccines and bacterial vectors are being explored as well, but are still at early stages of development for use against infectious pathogens. Whereas classic whole-organism vaccine platforms require the cultivation of the pathogen, next-generation viral vectored or nucleic acid-based vaccines can be constructed using the pathogen genetic sequence only, thereby significantly increasing the speed of development and manufacturing processes 125 .
Viral vectored vaccines are based on a recombinant virus (either replicating or not), in which the genome is altered to express the target pathogen antigen. The presentation of pathogen antigens in combination with stimuli from the viral vector that mimic natural infection leads to the induction of strong humoral and cellular immune responses without the need for an adjuvant. A potential disadvantage of viral vectored vaccines is the presence of pre-existing immunity when a vector such as human adenovirus is used that commonly causes infection in humans. This can be overcome by using vectors such as a simian adenovirus, against which almost no pre-existing immunity exists in humans 126 . Whether immune responses against the vector will limit its use for repeated vaccinations with different antigens will need to be investigated.
Nucleic acid-based vaccines consist of either DNA or RNA encoding the target antigen, which potentially allows for the induction of both humoral and cellular immune responses once the encoded antigens are expressed by the vaccine recipient after uptake of the nucleic acid by their cells. A huge advantage of these vaccines is that they are highly versatile and quick and easy to adapt and produce in the case of an emerging pathogen. Indeed, the SARS-CoV-2 mRNA-based vaccine mRNA-1273 entered clinical testing just 2 months after the genetic sequence of SARS-CoV-2 was identified 127 and the BNT162b2 lipid nanoparticle-formulated, nucleoside-modified RNA vaccine was the first SARS-CoV-2 vaccine to be licensed 128 . One of the disadvantages of these vaccines is that they need to be delivered directly into cells, which requires specific injection devices, electroporation or a carrier molecule and brings with it a risk of low transfection rate and limited immunogenicity 129 . Furthermore, the application of RNA vaccines has been limited by their lack of stability and requirement for a cold chain, but constant efforts to improve formulations hold promise to overcome these limitations 130 , 131 .
A beautiful example of how immunological insight can revolutionize vaccine development is the novel RSV vaccine DS-Cav1. The RSV surface fusion (F) protein can exist in either a pre-fusion (pre-F) conformation, which facilitates viral entry, or a post-fusion (post-F) form. Whereas previous vaccines mainly contained the post-F form, insight into the atomic-level structure of the protein has allowed for stable expression of the pre-F protein, leading to strongly enhanced immune responses and providing a proof of concept for structure-based vaccine design 132 , 133 .
In addition to the novel vaccine platforms mentioned above, there are ongoing efforts to develop improved methods of antigen delivery, such as liposomes (spherical lipid bilayers), polymeric particles, inorganic particles, outer membrane vesicles and immunostimulating complexes. These, and other methods such as self-assembling protein nanoparticles, have the potential to optimally enhance and skew the immune response to pathogens against which traditional vaccine approaches have proven to be unsuccessful 129 , 134 . Furthermore, innovative delivery methods, such as microneedle patches, are being developed, with the potential advantages of improved thermostability, ease of delivery with minimal pain and safer administration and disposal 135 . An inactivated influenza vaccine delivered by microneedle patch was shown to be well tolerated and immunogenic in a phase I trial 136 . This might allow for self-administration, although it would be important for professional medical care to be available if there is a risk of severe side effects such as anaphylaxis.
Conclusions and future directions
Immunization protects populations from diseases that previously claimed the lives of millions of individuals each year, mostly children. Under the United Nations Convention on the Rights of the Child, every child has the right to the best possible health, and by extrapolation a right to be vaccinated.
Despite the outstanding success of vaccination in protecting the health of our children, there are important knowledge gaps and challenges to be addressed. An incomplete understanding of immune mechanisms of protection and the lack of solutions to overcome antigenic variability have hampered the design of effective vaccines against major diseases such as HIV/AIDS and TB. Huge efforts have resulted in the licensure of a partially effective vaccine against malaria, but more effective vaccines will be needed to defeat this disease. Moreover, it is becoming clear that variation in host response is an important factor to take into account. New technologies and analytical methods will aid the delineation of the complex immune mechanisms involved, and this knowledge will be important to design effective vaccines for the future.
Apart from the scientific challenges, sociopolitical barriers stand in the way of safe and effective vaccination for all. Access to vaccines is one of the greatest obstacles, and improving infrastructure, continuing education and enhancing community engagement will be essential to improve this, and novel delivery platforms that eliminate the need for a cold chain could have great implications. There is a growing subset of the population who are sceptical about vaccination and this requires a response from the scientific community to provide transparency about the existing knowledge gaps and strategies to overcome these. Constructive collaboration between scientists and between scientific institutions, governments and industry will be imperative to move forwards. The COVID-19 pandemic has indeed shown that, in the case of an emergency, many parties with different incentives can come together to ensure that vaccines are being developed at unprecedented speed but has also highlighted some of the challenges of national and commercial interests. As immunologists, we have a responsibility to create an environment where immunization is normal, the science is accessible and robust, and access to vaccination is a right and expectation.
Change history
05 january 2021.
A Correction to this paper has been published: https://doi.org/10.1038/s41577-020-00497-5.
World Health Organization. Global vaccine action plan 2011–2020. WHO https://www.who.int/immunization/global_vaccine_action_plan/GVAP_doc_2011_2020/en/ (2013).
World Health Organization. Child mortality and causes of death. WHO https://www.who.int/gho/child_health/mortality/mortality_under_five_text/en/ (2020).
Hatherill, M., White, R. G. & Hawn, T. R. Clinical development of new TB vaccines: recent advances and next steps. Front. Microbiol. 10 , 3154 (2019).
Article PubMed Google Scholar
Bekker, L. G. et al. The complex challenges of HIV vaccine development require renewed and expanded global commitment. Lancet 395 , 384–388 (2020).
Matz, K. M., Marzi, A. & Feldmann, H. Ebola vaccine trials: progress in vaccine safety and immunogenicity. Expert Rev. Vaccines 18 , 1229–1242 (2019).
Article CAS PubMed Google Scholar
Ahmed, S. F., Quadeer, A. A. & McKay, M. R. Preliminary identification of potential vaccine targets for the COVID-19 coronavirus (SARS-CoV-2) based on SARS-CoV immunological studies. Viruses 12 , 254 (2020).
Article CAS PubMed Central Google Scholar
Pawelec, G. Age and immunity: what is “immunosenescence”? Exp. Gerontol. 105 , 4–9 (2018).
Larson, H. J. The state of vaccine confidence. Lancet 392 , 2244–2246 (2018).
Robbins, J. B. et al. Prevention of invasive bacterial diseases by immunization with polysaccharide–protein conjugates. Curr. Top. Microbiol. Immunol. 146 , 169–180 (1989).
CAS PubMed Google Scholar
Plotkin, S. A. Updates on immunologic correlates of vaccine-induced protection. Vaccine 38 , 2250–2257 (2020). This paper presents a review of immune correlates of protection for specific infections, their immunological basis and relevance for vaccinology .
Rubin, L. G. et al. 2013 IDSA clinical practice guideline for vaccination of the immunocompromised host. Clin. Infect. Dis. 58 , e44–e100 (2014).
Milligan, R., Paul, M., Richardson, M. & Neuberger, A. Vaccines for preventing typhoid fever. Cochrane Database Syst. Rev. 5 , CD001261 (2018).
PubMed Google Scholar
WHO. Measles vaccines: WHO position paper — April 2017. Wkly. Epidemiol. Rec. 92 , 205–227 (2017).
Google Scholar
Rappuoli, R., Mandl, C. W., Black, S. & De Gregorio, E. Vaccines for the twenty-first century society. Nat. Rev. Immunol. 11 , 865–872 (2011). This paper presents a review of the role of vaccines in the twenty-first century, with an emphasis on increased life expectancy, emerging infections and poverty .
Article CAS PubMed PubMed Central Google Scholar
Marrack, P., McKee, A. S. & Munks, M. W. Towards an understanding of the adjuvant action of aluminium. Nat. Rev. Immunol. 9 , 287–293 (2009).
Wilkins, A. L. et al. AS03- and MF59-adjuvanted influenza vaccines in children. Front. Immunol. 8 , 1760 (2017).
Article PubMed PubMed Central CAS Google Scholar
Kaslow, D. C. & Biernaux, S. RTS,S: toward a first landmark on the Malaria Vaccine Technology Roadmap. Vaccine 33 , 7425–7432 (2015).
Pedersen, C. et al. Immunization of early adolescent females with human papillomavirus type 16 and 18 L1 virus-like particle vaccine containing AS04 adjuvant. J. Adolesc. Health 40 , 564–571 (2007).
Mitkus, R. J., Hess, M. A. & Schwartz, S. L. Pharmacokinetic modeling as an approach to assessing the safety of residual formaldehyde in infant vaccines. Vaccine 31 , 2738–2743 (2013).
Eldred, B. E., Dean, A. J., McGuire, T. M. & Nash, A. L. Vaccine components and constituents: responding to consumer concerns. Med. J. Aust. 184 , 170–175 (2006).
Fijen, C. A., Kuijper, E. J., te Bulte, M. T., Daha, M. R. & Dankert, J. Assessment of complement deficiency in patients with meningococcal disease in The Netherlands. Clin. Infect. Dis. 28 , 98–105 (1999).
Wara, D. W. Host defense against Streptococcus pneumoniae : the role of the spleen. Rev. Infect. Dis. 3 , 299–309 (1981).
Gershon, A. A. et al. Varicella zoster virus infection. Nat. Rev. Dis. Prim. 1 , 15016 (2015).
Sandmann, F. et al. Infant hospitalisations and fatalities averted by the maternal pertussis vaccination programme in England, 2012–2017: post-implementation economic evaluation. Clin. Infect. Dis. 71 , 1984–1987 (2020).
Demicheli, V., Barale, A. & Rivetti, A. Vaccines for women for preventing neonatal tetanus. Cochrane Database Syst. Rev. 7 , CD002959 (2015).
Madhi, S. A. et al. Influenza vaccination of pregnant women and protection of their infants. N. Engl. J. Med. 371 , 918–931 (2014).
Article PubMed CAS Google Scholar
Madhi, S. A. & Dangor, Z. Prospects for preventing infant invasive GBS disease through maternal vaccination. Vaccine 35 , 4457–4460 (2017).
Madhi, S. A. et al. Respiratory syncytial virus vaccination during pregnancy and effects in infants. N. Engl. J. Med. 383 , 426–439 (2020).
Young, M. K. & Cripps, A. W. Passive immunization for the public health control of communicable diseases: current status in four high-income countries and where to next. Hum. Vaccin. Immunother. 9 , 1885–1893 (2013).
Patel, M. & Lee, C. K. Polysaccharide vaccines for preventing serogroup A meningococcal meningitis. Cochrane Database Syst. Rev. 3 , CD001093 (2005).
Moberley, S., Holden, J., Tatham, D. P. & Andrews, R. M. Vaccines for preventing pneumococcal infection in adults. Cochrane Database Syst. Rev. 1 , CD000422 (2013).
Andrews, N. J. et al. Serotype-specific effectiveness and correlates of protection for the 13-valent pneumococcal conjugate vaccine: a postlicensure indirect cohort study. Lancet Infect. Dis. 14 , 839–846 (2014).
Borrow, R., Abad, R., Trotter, C., van der Klis, F. R. & Vazquez, J. A. Effectiveness of meningococcal serogroup C vaccine programmes. Vaccine 31 , 4477–4486 (2013).
Ramsay, M. E., McVernon, J., Andrews, N. J., Heath, P. T. & Slack, M. P. Estimating haemophilus influenzae type b vaccine effectiveness in England and Wales by use of the screening method. J. Infect. Dis. 188 , 481–485 (2003).
Pollard, A. J., Perrett, K. P. & Beverley, P. C. Maintaining protection against invasive bacteria with protein-polysaccharide conjugate vaccines. Nat. Rev. Immunol. 9 , 213–220 (2009). This paper presents a review of the mechanism of action of polysaccharide vaccines and their role in establishing long-term protection against invasive bacteria .
Darton, T. C. et al. Design, recruitment, and microbiological considerations in human challenge studies. Lancet Infect. Dis. 15 , 840–851 (2015). This paper presents an overview of human challenge models, their potential use and their role in improving our understanding of disease mechanisms and host responses .
Suscovich, T. J. et al. Mapping functional humoral correlates of protection against malaria challenge following RTS, S/AS01 vaccination. Sci. Transl Med. 12 , eabb4757 (2020).
Jin, C. et al. Efficacy and immunogenicity of a Vi–tetanus toxoid conjugate vaccine in the prevention of typhoid fever using a controlled human infection model of Salmonella Typhi : a randomised controlled, phase 2b trial. Lancet 390 , 2472–2480 (2017).
Kourtis, A. P., Read, J. S. & Jamieson, D. J. Pregnancy and infection. N. Engl. J. Med. 370 , 2211–2218 (2014).
Malley, R. et al. CD4 + T cells mediate antibody-independent acquired immunity to pneumococcal colonization. Proc. Natl Acad. Sci. USA 102 , 4848–4853 (2005).
Henry, B. & Baclic, O. & National Advisory Committee on Immunization (NACI). Summary of the NACI update on the recommended use of hepatitis B vaccine. Can. Commun. Dis. Rep. 43 , 104–106 (2017).
Kelly, D. F., Pollard, A. J. & Moxon, E. R. Immunological memory: the role of B cells in long-term protection against invasive bacterial pathogens. JAMA 294 , 3019–3023 (2005).
McVernon, J., Johnson, P. D., Pollard, A. J., Slack, M. P. & Moxon, E. R. Immunologic memory in Haemophilus influenzae type b conjugate vaccine failure. Arch. Dis. Child. 88 , 379–383 (2003).
McVernon, J. et al. Immunologic memory with no detectable bactericidal antibody response to a first dose of meningococcal serogroup C conjugate vaccine at four years. Pediatr. Infect. Dis. J. 22 , 659–661 (2003).
World Health Organization. Tetanus vaccines: WHO position paper, February 2017 — recommendations. Vaccine 36 , 3573–3575 (2018).
Article Google Scholar
World Health Organization. Diphtheria vaccine: WHO position paper, August 2017 — recommendations. Vaccine 36 , 199–201 (2018).
Chen, Z. & He, Q. Immune persistence after pertussis vaccination. Hum. Vaccin. Immunother. 13 , 744–756 (2017).
Article PubMed PubMed Central Google Scholar
Burdin, N., Handy, L. K. & Plotkin, S. A. What is wrong with pertussis vaccine immunity? The problem of waning effectiveness of pertussis vaccines. Cold Spring Harb. Perspect. Biol. 9 , a029454 (2017).
WHO. Vaccines and vaccination against yellow fever: WHO Position Paper, June 2013 — recommendations. Vaccine 33 , 76–77 (2015).
Paunio, M. et al. Twice vaccinated recipients are better protected against epidemic measles than are single dose recipients of measles containing vaccine. J. Epidemiol. Community Health 53 , 173–178 (1999).
Zhu, S., Zeng, F., Xia, L., He, H. & Zhang, J. Incidence rate of breakthrough varicella observed in healthy children after 1 or 2 doses of varicella vaccine: results from a meta-analysis. Am. J. Infect. Control. 46 , e1–e7 (2018).
Halstead, S. B., Rojanasuphot, S. & Sangkawibha, N. Original antigenic sin in dengue. Am. J. Trop. Med. Hyg. 32 , 154–156 (1983).
Kim, J. H., Skountzou, I., Compans, R. & Jacob, J. Original antigenic sin responses to influenza viruses. J. Immunol. 183 , 3294–3301 (2009).
Vatti, A. et al. Original antigenic sin: a comprehensive review. J. Autoimmun. 83 , 12–21 (2017).
Statista Research Department. Herd immunity threshold for selected global diseases as of 2013. Statista https://www.statista.com/statistics/348750/threshold-for-herd-immunity-for-select-diseases/ (2013).
Plans-Rubio, P. The vaccination coverage required to establish herd immunity against influenza viruses. Prev. Med. 55 , 72–77 (2012).
Trotter, C. L., Andrews, N. J., Kaczmarski, E. B., Miller, E. & Ramsay, M. E. Effectiveness of meningococcal serogroup C conjugate vaccine 4 years after introduction. Lancet 364 , 365–367 (2004).
Trotter, C. L. & Maiden, M. C. Meningococcal vaccines and herd immunity: lessons learned from serogroup C conjugate vaccination programs. Expert. Rev. Vaccines 8 , 851–861 (2009).
Tabrizi, S. N. et al. Assessment of herd immunity and cross-protection after a human papillomavirus vaccination programme in Australia: a repeat cross-sectional study. Lancet Infect. Dis. 14 , 958–966 (2014).
Brisson, M. et al. Population-level impact, herd immunity, and elimination after human papillomavirus vaccination: a systematic review and meta-analysis of predictions from transmission-dynamic models. Lancet Public Health 1 , e8–e17 (2016).
Trunz, B. B., Fine, P. & Dye, C. Effect of BCG vaccination on childhood tuberculous meningitis and miliary tuberculosis worldwide: a meta-analysis and assessment of cost-effectiveness. Lancet 367 , 1173–1180 (2006).
Barker, L. & Hussey, G. The Immunological Basis for Immunization Series: Module 5: Tuberculosis (World Health Organization, 2011).
Eisenhut, M. et al. BCG vaccination reduces risk of infection with Mycobacterium tuberculosis as detected by γ interferon release assay. Vaccine 27 , 6116–6120 (2009).
Verrall, A. J. et al. Early clearance of Mycobacterium tuberculosis : the INFECT case contact cohort study in Indonesia. J. Infect. Dis. 221 , 1351–1360 (2020).
Pollard, A. J., Finn, A. & Curtis, N. Non-specific effects of vaccines: plausible and potentially important, but implications uncertain. Arch. Dis. Child. 102 , 1077–1081 (2017).
Higgins, J. P. et al. Association of BCG, DTP, and measles containing vaccines with childhood mortality: systematic review. BMJ 355 , i5170 (2016).
Mina, M. J. et al. Measles virus infection diminishes preexisting antibodies that offer protection from other pathogens. Science 366 , 599–606 (2019).
Mina, M. J., Metcalf, C. J., de Swart, R. L., Osterhaus, A. D. & Grenfell, B. T. Long-term measles-induced immunomodulation increases overall childhood infectious disease mortality. Science 348 , 694–699 (2015). This paper is an analysis of population-level data from high-income countries, showing a protective effect of measles vaccination on mortality from non-measles infectious diseases .
Falsey, A. R., Treanor, J. J., Tornieporth, N., Capellan, J. & Gorse, G. J. Randomized, double-blind controlled phase 3 trial comparing the immunogenicity of high-dose and standard-dose influenza vaccine in adults 65 years of age and older. J. Infect. Dis. 200 , 172–180 (2009).
DiazGranados, C. A. et al. Efficacy of high-dose versus standard-dose influenza vaccine in older adults. N. Engl. J. Med. 371 , 635–645 (2014).
Schnyder, J. L. et al. Fractional dose of intradermal compared to intramuscular and subcutaneous vaccination—a systematic review and meta-analysis. Travel. Med. Infect. Dis. 37 , 101868 (2020).
Voysey, M. et al. The influence of maternally derived antibody and infant age at vaccination on infant vaccine responses: an individual participant meta-analysis. JAMA Pediatr. 171 , 637–646 (2017).
Caceres, V. M., Strebel, P. M. & Sutter, R. W. Factors determining prevalence of maternal antibody to measles virus throughout infancy: a review. Clin. Infect. Dis. 31 , 110–119 (2000).
Belnoue, E. et al. APRIL is critical for plasmablast survival in the bone marrow and poorly expressed by early-life bone marrow stromal cells. Blood 111 , 2755–2764 (2008).
Pace, D. et al. Immunogenicity of reduced dose priming schedules of serogroup C meningococcal conjugate vaccine followed by booster at 12 months in infants: open label randomised controlled trial. BMJ 350 , h1554 (2015).
Timens, W., Boes, A., Rozeboom-Uiterwijk, T. & Poppema, S. Immaturity of the human splenic marginal zone in infancy. Possible contribution to the deficient infant immune response. J. Immunol. 143 , 3200–3206 (1989).
Peset Llopis, M. J., Harms, G., Hardonk, M. J. & Timens, W. Human immune response to pneumococcal polysaccharides: complement-mediated localization preferentially on CD21-positive splenic marginal zone B cells and follicular dendritic cells. J. Allergy Clin. Immunol. 97 , 1015–1024 (1996).
Claesson, B. A. et al. Protective levels of serum antibodies stimulated in infants by two injections of Haemophilus influenzae type b capsular polysaccharide–tetanus toxoid conjugate. J. Pediatr. 114 , 97–100 (1989).
Crooke, S. N., Ovsyannikova, I. G., Poland, G. A. & Kennedy, R. B. Immunosenescence and human vaccine immune responses. Immun. Ageing 16 , 25 (2019).
Nikolich-Žugich, J. Ageing and life-long maintenance of T-cell subsets in the face of latent persistent infections. Nat. Rev. Immunol. 8 , 512–522 (2008).
Kadambari, S., Klenerman, P. & Pollard, A. J. Why the elderly appear to be more severely affected by COVID-19: the potential role of immunosenescence and CMV. Rev. Med. Virol. 30 , e2144 (2020).
Domnich, A. et al. Effectiveness of MF59-adjuvanted seasonal influenza vaccine in the elderly: a systematic review and meta-analysis. Vaccine 35 , 513–520 (2017).
Lal, H. et al. Efficacy of an adjuvanted herpes zoster subunit vaccine in older adults. N. Engl. J. Med. 372 , 2087–2096 (2015).
World Health Assembly. The Expanded Programme on Immunization: the 1974 resolution by the World Health Assembly. Assign. Child. 69-72 , 87–88 (1985).
Voysey, M., Pollard, A. J., Sadarangani, M. & Fanshawe, T. R. Prevalence and decay of maternal pneumococcal and meningococcal antibodies: a meta-analysis of type-specific decay rates. Vaccine 35 , 5850–5857 (2017).
Farrington, P. et al. A new method for active surveillance of adverse events from diphtheria/tetanus/pertussis and measles/mumps/rubella vaccines. Lancet 345 , 567–569 (1995).
Pinto, M. V., Bihari, S. & Snape, M. D. Immunisation of the immunocompromised child. J. Infect. 72 (Suppl), S13–S22 (2016).
Seligman, S. J. Risk groups for yellow fever vaccine-associated viscerotropic disease (YEL-AVD). Vaccine 32 , 5769–5775 (2014).
Gellin, B. G., Maibach, E. W. & Marcuse, E. K. Do parents understand immunizations? A national telephone survey. Pediatrics 106 , 1097–1102 (2000).
Offit, P. A. et al. Addressing parents’ concerns: do multiple vaccines overwhelm or weaken the infant’s immune system? Pediatrics 109 , 124–129 (2002).
Centers for Disease Control and Prevention. Multiple vaccinations at once. CDC https://www.cdc.gov/vaccinesafety/concerns/multiple-vaccines-immunity.html (2020).
Stowe, J., Andrews, N., Taylor, B. & Miller, E. No evidence of an increase of bacterial and viral infections following measles, mumps and rubella vaccine. Vaccine 27 , 1422–1425 (2009).
Otto, S. et al. General non-specific morbidity is reduced after vaccination within the third month of life — the Greifswald study. J. Infect. 41 , 172–175 (2000).
Griffin, M. R., Taylor, J. A., Daugherty, J. R. & Ray, W. A. No increased risk for invasive bacterial infection found following diphtheria–tetanus–pertussis immunization. Pediatrics 89 , 640–642 (1992).
Aaby, P. et al. Non-specific beneficial effect of measles immunisation: analysis of mortality studies from developing countries. BMJ 311 , 481–485 (1995).
Glanz, J. M. et al. Association between estimated cumulative vaccine antigen exposure through the first 23 months of life and non-vaccine-targeted infections from 24 through 47 months of age. JAMA 319 , 906–913 (2018).
Bohlke, K. et al. Risk of anaphylaxis after vaccination of children and adolescents. Pediatrics 112 , 815–820 (2003).
Nohynek, H. et al. AS03 adjuvanted AH1N1 vaccine associated with an abrupt increase in the incidence of childhood narcolepsy in Finland. PLoS ONE 7 , e33536 (2012).
Miller, E. et al. Risk of narcolepsy in children and young people receiving AS03 adjuvanted pandemic A/H1N1 2009 influenza vaccine: retrospective analysis. BMJ 346 , f794 (2013).
Hallberg, P. et al. Pandemrix-induced narcolepsy is associated with genes related to immunity and neuronal survival. EBioMedicine 40 , 595–604 (2019).
DeStefano, F. & Shimabukuro, T. T. The MMR vaccine and autism. Annu. Rev. Virol. 6 , 585–600 (2019).
DeStefano, F., Bodenstab, H. M. & Offit, P. A. Principal controversies in vaccine safety in the United States. Clin. Infect. Dis. 69 , 726–731 (2019).
Moro, P. L., Haber, P. & McNeil, M. M. Challenges in evaluating post-licensure vaccine safety: observations from the Centers for Disease Control and Prevention. Expert Rev. Vaccines 18 , 1091–1101 (2019).
Peck, M. et al. Global routine vaccination coverage, 2018. MMWR Morb. Mortal. Wkly. Rep. 68 , 937–942 (2019).
World Health Organization. Immunization coverage. WHO https://www.who.int/news-room/fact-sheets/detail/immunization-coverage (2020).
World Health Organization. More than 9.4 million children vaccinated against typhoid fever in Sindh. WHO http://www.emro.who.int/pak/pakistan-news/more-than-94-children-vaccinated-with-typhoid-conjugate-vaccine-in-sindh.html (2019).
World Health Organization. More than 140,000 die from measles as cases surge worldwide. WHO https://www.who.int/news-room/detail/05-12-2019-more-than-140-000-die-from-measles-as-cases-surge-worldwide (2019).
World Health Organization. Disease outbreaks. WHO https://www.who.int/emergencies/diseases/en/ (2020).
Rerks-Ngarm, S. et al. Vaccination with ALVAC and AIDSVAX to prevent HIV-1 infection in Thailand. N. Engl. J. Med. 361 , 2209–2220 (2009).
Fauci, A. S., Marovich, M. A., Dieffenbach, C. W., Hunter, E. & Buchbinder, S. P. Immunology. Immune activation with HIV vaccines. Science 344 , 49–51 (2014).
Agnandji, S. T. et al. A phase 3 trial of RTS,S/AS01 malaria vaccine in African infants. N. Engl. J. Med. 367 , 2284–2295 (2012).
Killeen, G. F. et al. Developing an expanded vector control toolbox for malaria elimination. BMJ Glob. Health 2 , e000211 (2017).
Osterholm, M. T., Kelley, N. S., Sommer, A. & Belongia, E. A. Efficacy and effectiveness of influenza vaccines: a systematic review and meta-analysis. Lancet Infect. Dis. 12 , 36–44 (2012).
Skowronski, D. M. et al. Low 2012–13 influenza vaccine effectiveness associated with mutation in the egg-adapted H3N2 vaccine strain not antigenic drift in circulating viruses. PLoS ONE 9 , e92153 (2014).
Raymond, D. D. et al. Conserved epitope on influenza-virus hemagglutinin head defined by a vaccine-induced antibody. Proc. Natl Acad. Sci. USA 115 , 168–173 (2018).
Tameris, M. D. et al. Safety and efficacy of MVA85A, a new tuberculosis vaccine, in infants previously vaccinated with BCG: a randomised, placebo-controlled phase 2b trial. Lancet 381 , 1021–1028 (2013).
Tait, D. R. et al. Final analysis of a trial of M72/AS01(E) vaccine to prevent tuberculosis. N. Engl. J. Med. 381 , 2429–2439 (2019).
Kobayashi, M. et al. WHO consultation on group B streptococcus vaccine development: report from a meeting held on 27–28 April 2016. Vaccine 37 , 7307–7314 (2019).
Inoue, N., Abe, M., Kobayashi, R. & Yamada, S. Vaccine development for cytomegalovirus. Adv. Exp. Med. Biol. 1045 , 271–296 (2018).
Schleiss, M. R., Permar, S. R. & Plotkin, S. A. Progress toward development of a vaccine against congenital cytomegalovirus infection. Clin. Vaccine Immunol. 24 , e00268–e00317 (2017).
CAS PubMed PubMed Central Google Scholar
World Health Organization. Ageing and health. WHO https://www.who.int/news-room/fact-sheets/detail/ageing-and-health (2018).
Rauch, S., Jasny, E., Schmidt, K. E. & Petsch, B. New vaccine technologies to combat outbreak situations. Front. Immunol. 9 , 1963 (2018).
Jeyanathan, M. et al. Immunological considerations for COVID-19 vaccine strategies. Nat. Rev. Immunol. 20 , 615–632 (2020). This paper is an overview of COVID-19 vaccine development, with emphasis on underlying immunological mechanisms and potential scenarios for global development .
Koff, W. C. & Schenkelberg, T. The future of vaccine development. Vaccine 38 , 4485–4486 (2020).
van Riel, D. & de Wit, E. Next-generation vaccine platforms for COVID-19. Nat. Mater. 19 , 810–812 (2020).
Rollier, C. S., Reyes-Sandoval, A., Cottingham, M. G., Ewer, K. & Hill, A. V. Viral vectors as vaccine platforms: deployment in sight. Curr. Opin. Immunol. 23 , 377–382 (2011).
Corbett, K. S. et al. SARS-CoV-2 mRNA vaccine design enabled by prototype pathogen preparedness. Nature 586 , 567–571 (2020).
Polack, F. P. et al. Safety and efficacy of the BNT162b2 mRNA Covid-19 vaccine. N. Engl. J. Med. https://doi.org/10.1056/NEJMoa2034577 (2020).
Wallis, J., Shenton, D. P. & Carlisle, R. C. Novel approaches for the design, delivery and administration of vaccine technologies. Clin. Exp. Immunol. 196 , 189–204 (2019).
Zhang, C., Maruggi, G., Shan, H. & Li, J. Advances in mRNA vaccines for infectious diseases. Front. Immunol. 10 , 594 (2019).
Pardi, N., Hogan, M. J., Porter, F. W. & Weissman, D. mRNA vaccines — a new era in vaccinology. Nat. Rev. Drug Discov. 17 , 261–279 (2018).
Crank, M. C. et al. A proof of concept for structure-based vaccine design targeting RSV in humans. Science 365 , 505–509 (2019). This paper presents a phase I trial demonstrating enhanced immunogenicity of the pre-F conformation of RSV surface protein, thereby providing a proof of concept for successful structure-based vaccine design .
Mascola, J. R. & Fauci, A. S. Novel vaccine technologies for the 21st century. Nat. Rev. Immunol. 20 , 87–88 (2020).
Kanekiyo, M., Ellis, D. & King, N. P. New vaccine design and delivery technologies. J. Infect. Dis. 219 , S88–S96 (2019).
Peyraud, N. et al. Potential use of microarray patches for vaccine delivery in low- and middle-income countries. Vaccine 37 , 4427–4434 (2019).
Rouphael, N. G. et al. The safety, immunogenicity, and acceptability of inactivated influenza vaccine delivered by microneedle patch (TIV-MNP 2015): a randomised, partly blinded, placebo-controlled, phase 1 trial. Lancet 390 , 649–658 (2017).
Davenport, R. J., Satchell, M. & Shaw-Taylor, L. M. W. The geography of smallpox in England before vaccination: a conundrum resolved. Soc. Sci. Med. 206 , 75–85 (2018).
Download references
Acknowledgements
The authors thank all those whose work in the development, policy and delivery of vaccines underpins immunization programmes to defend our health and the health of our children.
Author information
Authors and affiliations.
Oxford Vaccine Group, Department of Paediatrics, University of Oxford, Oxford, UK
Andrew J. Pollard & Else M. Bijker
NIHR Oxford Biomedical Research Centre, Oxford University Hospitals Trust, Oxford, UK
You can also search for this author in PubMed Google Scholar
Contributions
The authors contributed equally to all aspects of the article.
Corresponding author
Correspondence to Andrew J. Pollard .
Ethics declarations
Competing interests.
A.J.P. is Chair of the UK Department of Health and Social Care’s (DHSC) Joint Committee on Vaccination and Immunisation (JCVI), a member of the World Health Organization (WHO) Strategic Advisory Group of Experts on Immunization (SAGE) and a National Institute for Health Research (NIHR) Senior Investigator. The views expressed in this article do not necessarily represent the views of the DHSC, JCVI, NIHR or WHO. E.M.B. declares no competing interests. Oxford University has entered into a partnership with AstraZeneca for the development of a viral vectored coronavirus vaccine.
Additional information
Peer review information.
Nature Reviews Immunology thanks the anonymous reviewers for their contribution to the peer review of this work.
Publisher’s note
Springer Nature remains neutral with regard to jurisdictional claims in published maps and institutional affiliations.
Related links
Advisory Committee on Immunization Practices (ACIP): https://www.cdc.gov/vaccines/acip/index.html
Coalition for Epidemic Preparedness Innovations (CEPI): https://cepi.net/
Gavi, the Vaccine Alliance: https://www.gavi.org/
Joint Committee on Vaccination and Immunisation (JCVI): https://www.gov.uk/government/groups/joint-committee-on-vaccination-and-immunisation
Nature Milestones in Vaccines: https://www.nature.com/immersive/d42859-020-00005-8/index.html
The Green Book, information for public health professionals on immunisation, Public Health England : https://www.gov.uk/government/collections/immunisation-against-infectious-disease-the-green-book
Vaccine Knowledge Project: https://vk.ovg.ox.ac.uk/vk/
Vaccines 101: How new vaccines are developed: https://www.youtube.com/watch?v=2t_mQwTY4WQ&feature=emb_logo
Vaccines 101: How vaccines work: https://www.youtube.com/watch?v=4SKmAlQtAj8&feature=emb_logo
Supplementary information
Supplementary information.
Parts of the pathogen (such as proteins or polysaccharides) that are recognized by the immune system and can be used to induce an immune response by vaccination.
The state in which an individual does not develop disease after being exposed to a pathogen.
A reduction in the virulence of a pathogen (through either deliberate or natural changes in virulence genes).
Particles constructed of viral proteins that structurally mimic the native virus but lack the viral genome.
An agent used in a vaccine to enhance the immune response against the antigen.
Molecules that stimulate a more robust immune response together with an antigen. Endogenous mediators that are released in response to infection or injury and that interact with pattern recognition receptors such as Toll-like receptors to activate innate immune cells such as dendritic cells.
The evolutionarily primitive part of the immune system that detects foreign antigens in a non-specific manner.
A liposome-based adjuvant containing 3- O -desacyl-4′-monophosphoryl lipid A and the saponin QS-21. AS01 triggers the innate immune system immediately after vaccination, resulting in an enhanced adaptive immune response.
An adjuvant consisting of aluminium salt and the Toll-like receptor agonist monophosphoryl lipid A.
A network of proteins that form an important part of the immune response by enhancing the opsonization of pathogens, cell lysis and inflammation.
A state of a pathogen in which antibodies or complement factors are bound to its surface.
Antibodies that bind to a pathogen, which subsequently can be eliminated by phagocytosis.
Antigens against which B cells can mount an antibody response without T cell help.
An antigen for which T cell help is required in order for B cells to mount an antibody response.
Studies in which volunteers are deliberately infected with a pathogen, in a carefully conducted study, to evaluate the biology of infection and the efficacy of drugs and vaccines.
The capacity of the immune system to respond quicker and more effectively when a pathogen is encountered again after an initial exposure that induced antigen-specific B cells and T cells.
The period from acquisition of a pathogen to the development of symptomatic disease.
Repeat administration of a vaccine after an initial priming dose, given in order to enhance the immune response.
An assay in which blood is stimulated with Mycobacterium tuberculosis antigens, after which levels of interferon-γ (produced by specific memory T cells if these are present) are measured.
Changes in the expression of genes that do not result from changes in DNA sequence.
A severe and potentially life-threatening reaction to an allergen.
Vaccines that are administered by means avoiding the gastrointestinal tract (for example, by intramuscular, subcutaneous or intradermal routes).
An acquired autoimmune condition characterized by low levels of platelets in the blood caused by antibodies to platelet antigens.
A rare chronic sleep disorder characterized by extreme sleepiness during the day and sudden sleep attacks.
Vaccines that are intended for a limited scope or targeting infections that are rare, as a result of which development costs exceed their market potential.
Blebs made from the outer membrane of Gram-negative bacteria, containing the surface proteins and lipids of the organism in the membrane.
Rights and permissions
Reprints and permissions
About this article
Cite this article.
Pollard, A.J., Bijker, E.M. A guide to vaccinology: from basic principles to new developments. Nat Rev Immunol 21 , 83–100 (2021). https://doi.org/10.1038/s41577-020-00479-7
Download citation
Accepted : 19 November 2020
Published : 22 December 2020
Issue Date : February 2021
DOI : https://doi.org/10.1038/s41577-020-00479-7
Share this article
Anyone you share the following link with will be able to read this content:
Sorry, a shareable link is not currently available for this article.
Provided by the Springer Nature SharedIt content-sharing initiative
This article is cited by
Immunomodulatory effects of epiphytic loranthus micranthus leaf extracts collected from two host plants: psidium guajava and parkia biglobosa.
- Ngozi Dorathy Idoko
- Ifeoma Felicia Chukwuma
- Michel de Waard
BMC Complementary Medicine and Therapies (2024)
Ontological representation, modeling, and analysis of parasite vaccines
- Anthony Huffman
- Xumeng Zhang
Journal of Biomedical Semantics (2024)
Efficacy, immunogenicity and safety of respiratory syncytial virus prefusion F vaccine: systematic review and meta-analysis
BMC Public Health (2024)
Immunoinformatics design of a structural proteins driven multi-epitope candidate vaccine against different SARS-CoV-2 variants based on fynomer
- Javad Sarvmeili
- Bahram Baghban Kohnehrouz
- Hamideh Ofoghi
Scientific Reports (2024)
T-cell stimulating vaccines empower CD3 bispecific antibody therapy in solid tumors
- Jim Middelburg
- Marjolein Sluijter
- Thorbald van Hall
Nature Communications (2024)
Quick links
- Explore articles by subject
- Guide to authors
- Editorial policies
Sign up for the Nature Briefing: Microbiology newsletter — what matters in microbiology research, free to your inbox weekly.

- Systematic Review
- Open access
- Published: 06 May 2024
Efficacy, immunogenicity and safety of respiratory syncytial virus prefusion F vaccine: systematic review and meta-analysis
- Yi Pang 1 na1 ,
- Haishan Lu 2 , 3 na1 ,
- Demin Cao 2 , 3 ,
- Xiaoying Zhu 2 , 3 ,
- Qinqin Long 2 , 3 ,
- Fengqin Tian 2 , 3 ,
- Xidai Long 2 , 3 &
- Yulei Li 2 , 3
BMC Public Health volume 24 , Article number: 1244 ( 2024 ) Cite this article
356 Accesses
Metrics details
A notable research gap exists in the systematic review and meta-analysis concerning the efficacy, immunogenicity, and safety of the respiratory syncytial virus (RSV) prefusion F vaccine.
We conducted a comprehensive search across PubMed, Embase, the Cochrane Central Register of Controlled Trials, and ClinicalTrials.gov to retrieve articles related to the efficacy, immunogenicity, and safety of RSV prefusion F vaccines, published through September 8, 2023. We adhered to the Preferred Reporting Items for Systematic Reviews and Meta-Analyses guidelines.
A total of 22 randomized controlled trials involving 78,990 participants were included in this systematic review and meta-analysis. The RSV prefusion F vaccine exhibited a vaccine effectiveness of 68% (95% CI: 59–75%) against RSV-associated acute respiratory illness, 70% (95% CI: 60–77%) against medically attended RSV-associated lower respiratory tract illness, and 87% (95% CI: 71–94%) against medically attended severe RSV-associated lower respiratory tract illness. Common reported local adverse reactions following RSV prefusion F vaccination include pain, redness, and swelling at the injection site, and systemic reactions such as fatigue, headache, myalgia, arthralgia, nausea, and chills.
Conclusions
Our meta-analysis suggests that vaccines using the RSV prefusion F protein as antigen exhibit appears broadly acceptable efficacy, immunogenicity, and safety in the population. In particular, it provides high protective efficiency against severe RSV-associated lower respiratory tract disease.
Peer Review reports
Introduction
Respiratory syncytial virus (RSV), discovered in 1956, is a negative-sense single-stranded RNA virus belonging to the Pneumonaviridae family. RSV is highly contagious and represents a major burden of respiratory disease worldwide, causing severe and even fatal respiratory infections and bronchiolitis, especially in the elderly (≥ 65 years), young children (< 5 years), and those with underlying chronic diseases (e.g., pulmonary and circulatory diseases) [ 1 ]. In 2019, globally, there were 33 million events of RSV-associated acute lower respiratory tract infection (uncertainty range, 2.54 to 446 million) and 1.01 million total RSV-attributable deaths (84 500 to 125 200) in young children [ 2 ].
There has been a long road with multiple obstacles to developing a safe and effective RSV vaccine. Earlier vaccines provided insufficient protection as they used the post-F conformation as the vaccine antigen. This is because multiple unique antigenic sites are exposed on the surface of the F protein before RSV fuses with the host cell membrane. Following fusion, the F protein adopts a very different confirmation in which several antigenic sites are no longer exposed [ 3 ]. Thus, the stabilization of the pre-F conformation has made it possible to develop effective subunit vaccines [ 4 ]. On May 3, 2023, the U.S. Food and Drug Administration (FDA) approved the world’s first RSV vaccine (developed by GSK) and on May 31, 2023, the Pfizer vaccine, both for adults older than 60 years of age. Both vaccines use a prefusion stable variant of the F protein. RSV prefusion F vaccine has become a hot spot in the research of vaccines against RSV. A large number of clinical studies have investigated its protective efficacy. However, to date, no systematic reviews have been performed on the efficacy, immunogenicity and safety of RSV prefusion F vaccine. In this review, we compared the protective efficacy, antibody titer levels, and adverse reaction profiles of different RSV prefusion F vaccines between immunized individuals and controls.
This systematic review adheres to the Preferred Reporting Items for Systematic Reviews and Meta-Analyses (PRISMA) guidelines [ 5 ].
Search strategy
In September 2023, in accordance with the study protocol, we conducted searches across several databases, including Medline via PubMed, Embase, Cochrane Central Register of Controlled Trials (CENTRAL), and ClinicalTrials.gov, to identify articles published up to September 8, 2023. The following MeSH (Medical Subject Heading) terms and search terms were used: (“Respiratory Syncytial Viruses or RSV”) AND (“vaccine or vaccination or efficacy or adverse event”).
Eligibility criteria
The inclusion criteria included: (1) individual study populations being at least twenty cases; (2) the use of prefusion F protein as an immunogen is explicitly stated; (3) clinical trials in human subjects have been published. No language restrictions were imposed on the publications. To enhance the validity of the data, we excluded non-peer-reviewed articles from preprint databases.
Study selection
In this review, we employed a two-stage approach for screening, initially assessing titles and abstracts followed by full-text articles. Two researchers independently reviewed each title, abstract, and full text, with any discrepancies resolved through consensus with a third researcher. The efficacy of the vaccines were assessed on three endpoints. First, the efficacy of the vaccine in preventing RSV-associated acute respiratory illness which was defined as the ability of the vaccine to prevent RT-PCR-confirmed RSV infection within seven days of acute respiratory illness symptom onset. Second, the efficacy of the vaccine in preventing medically attended RSV-associated lower respiratory tract illness which was defined as at least two symptoms or signs of acute respiratory infection lasting at least 24 h (cough, abnormal breathing, fever, lethargy, or any other respiratory symptom of concern). Third, the efficacy of the vaccine in preventing medically attended severe RSV-associated lower respiratory tract illness which was defined as tachypnea (respiratory rate ≥ 70 breaths per minute in infants younger than two months [60 days] of age or ≥ 60 breaths per minute in those between two months and 12 months of age); SpO2 < 93% while the infant was breathing ambient air; use of oxygen delivered through a high-flow nasal cannula or mechanical ventilation; admission to an intensive care unit for more than 4 h; and unresponsiveness or unconsciousness. The efficacy of the RSV vaccine was based on assessing its efficacy during the first RSV season (about 6 months) after vaccination. All the efficacy endpoints were considered if they occurred at least seven days after the full regimen of the vaccine.
Data extraction
Two researchers extracted data using a predefined extraction form. Subsequently, all key extracted data underwent review and quality checking by the same two researchers at the conclusion of the data extraction phase. Data on study characteristics encompassed information regarding the setting, primary and secondary outcomes, study design, sample size, and exclusion and inclusion criteria. Participant data included details such as sex, age, and relevant medical history, including disease and treatment history. Intervention-related data consisted of the vaccine type and brand, dosing schedule, the number of participants receiving each type and brand of vaccine, and the median or mean interval between doses. Data pertaining to immunogenicity results included details such as the assay type, the specific antibody measured, T cell response, the method of measurement, intervals of sample collection, and the number of measurements conducted.
Risk of bias assessment
Two investigators independently evaluated the risk of bias in the included studies based on critical criteria, including random sequence generation, allocation concealment, blinding of participants, personnel, and outcomes, incomplete outcome data, selective outcome reporting, and other potential sources of bias, following the methods recommended by The Cochrane Collaboration. The risk of bias graph was generated using Revman 5.4 software. The following judgments were used: low risk, high risk, or unclear. Authors resolved disagreements by consensus and further article review if necessary.
Data analysis
We used RevMan 5.4 statistical software to pool dichotomous outcomes, with the risk ratio (RR) and its 95% confidence interval (CI) as the effect measures. RR < 1 implies a lower risk in the vaccinated group compared to the control group, and P < 0.05 indicates that this difference is statistically significant. The I 2 statistic was used to estimate the level of heterogeneity, and significant heterogeneity was considered when the I 2 value was > 50%. Vaccine efficacy was calculated using the fixed effects RR. This study applied the accepted statistical vaccine efficacy formula, (1 − RR) ×100, for calculating the pooled vaccine efficacy from the pooled RR. We conducted visual examinations of funnel plots and utilized Egger’s test to assess potential publication bias. Additionally, we employed the trim-and-fill analysis to evaluate the effect of publication bias on the pooled effect size estimates. Influence analysis, which constitutes a form of sensitivity analysis, was performed to identify the impact of individual studies on the combined estimates.
Study selection and study characteristics
A total of 10,554 records were initially retrieved from the database. After screening titles and abstracts, we evaluated 298 full texts of potentially eligible reports; a total of 22 articles were included, involving 78,990 participants (Fig. 1 ) [ 6 , 7 , 8 , 9 , 10 , 11 , 12 , 13 , 14 , 15 , 16 , 17 , 18 , 19 , 20 , 21 , 22 , 23 , 24 , 25 , 26 , 27 ]. Of the 22 eligible studies, eight (36%) studies were analyzed to evaluate the efficacy of RSV prefusion F vaccines, 20 (91%) studies were analyzed to evaluate immunogenicity, and 22 (100%) studies were analyzed to evaluate safety (Table 1 ). The included studies reported data for four vaccine types: 15 (68%) for subunit vaccines, five (23%) for adenovirus vaccines, one (4%) for mixed adenovirus and subunit vaccines, and one (4%) for mRNA vaccines. The 22 included studies involved diverse populations, with 10 involving older adults over 60 years of age, 4 involving pregnant women, 3 involving non-pregnant women, and 7 involving healthy adults. The included studies involved more than 20 countries or regions, with 11 (50%) studies being multinational, six (27%) studies from Spain, followed by two (9%) studies from Australia, and one each from Japan, Canada, and the United Kingdom. 12 (55%) of the eligible studies were observer-blinded and 10 (45%) were double-blinded.
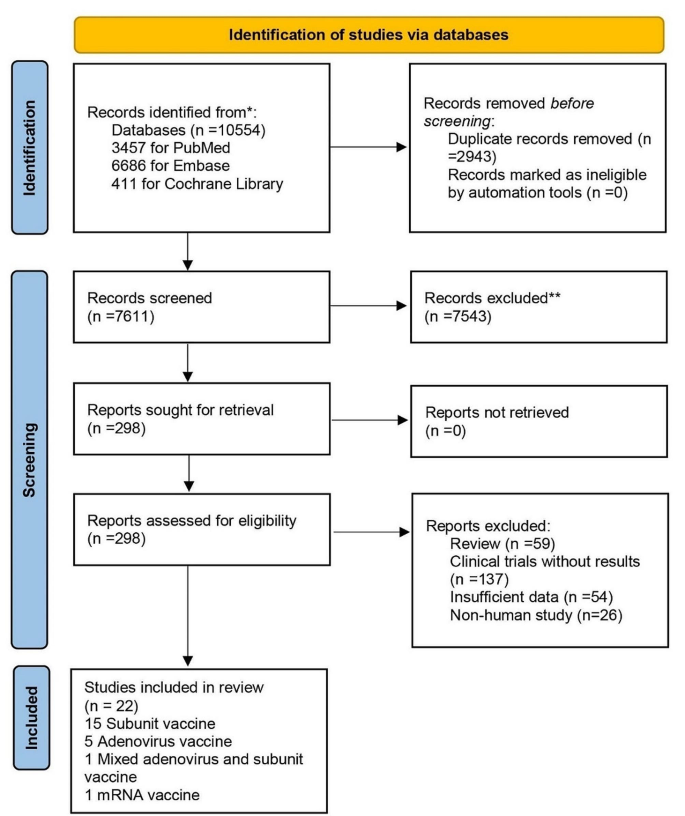
Flowchart of study selection
Risk of bias assessment of included studies
Twenty-two studies used Cochrane collaboration tools for independent risk of bias assessment, only two studies had high risk in blinding of outcome assessment, and most studies were low risk in all evaluated domains (Fig. 2 ). Overall, all of these included studies had a low risk of bias, with blinding and other biases in outcome assessment being the main risk factors.
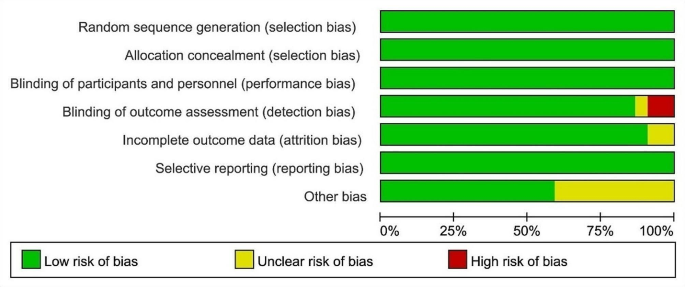
Risk of bias graph: review authors’ judgements about each risk of bias item presented as percentages across all included studies
Efficacy of RSV prefusion vaccine
Six (27%) studies were included to evaluate the efficacy of RSV prefusion vaccine in the prevention of RSV-associated acute respiratory illness. Data from 31,645 vaccinated patients compared with 31,672 controls showed a significant pooled risk reduction in the vaccinated group, with a RR of 0.32 (95% CI: 0.25 to 0.41, I 2 = 1%) and an overall vaccine efficacy of 68% (95% CI: 59–75%) (Fig. 3 ). A total of seven (32%) studies assessing the efficacy of vaccination against medically attended RSV-associated lower respiratory tract illness with data from 35,521 vaccinated versus 35,243 controls showed similarly significant pooled risk reductions in vaccinated groups, with a RR of 0.30 (RR 0.30, 95% CI: 0.23 to 0.40, I 2 = 22%). Three (14%) studies reported the lowest RR (RR 0.13, 95% CI: 0.06 to 0.29, I 2 = 0%) and minimal heterogeneity in severe RSV-associated lower respiratory tract illness requiring medical attention in the group that received the RSV prefusion F vaccines, with an overall vaccine efficacy of 87% (95% CI: 71–94%). When sensitivity analyses were performed, the heterogeneity of the pooled effects of the results did not change substantially after retaining only subunit vaccines, indicating that our results are robust and reliable.
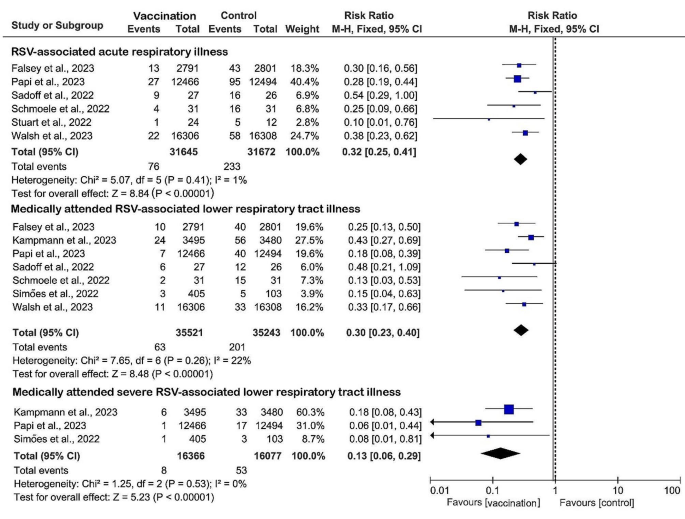
Vaccine efficacy compared with placebo calculated using the Mantel–Haenszel fixed effects model
Immunogenicity of RSV prefusion vaccine
Following the inclusion criteria, 20 studies (91%) on the immunogenicity of RSV prefusion F vaccines were included in this systematic review article (Table 2 ). There was a significant increase in neutralizing antibody titers against RSV-A in all studies, with a maximum increase of more than 20-fold from baseline. The neutralizing antibody titer against RSV-B was also significantly increased at about one month after immunization, with an increase of more than 1.4-fold compared with baseline. Seven studies examined T cell responses after vaccine immunization simultaneously, and the results showed that mixed adenovirus and subunit vaccine produced the strongest cellular immune responses, with up to 13-fold increase in interferon-γ secretion compared with baseline.
Safety of RSV prefusion vaccine
The safety profiles of 22 studies were reviewed, and adverse effects of RSV prefusion F vaccination included local reactions such as pain, redness, and swelling at the vaccination site and systemic reactions such as fatigue, headache, Myalgia, joint pain, nausea, and chills (Table 3 ). The subunit vaccine had the lowest risk of local and systemic adverse reactions, with RR of 2.79 (95% CI: 1.47 to 6.00, I 2 = 77%) and 1.24 (95% CI: 0.95 to 1.63, I 2 = 74%), respectively, and the risk of serious adverse events (grade ≥ 3) was also the lowest (RR 2.11, 95% CI: 1.41 to 3.15, I 2 = 25%) (Fig. 4 ; Table 3 ). Redness was the predominant local reaction observed among recipients of the subunit vaccine (RR 4.77, 95% CI: 3.08 to 7.38, I 2 = 41%). Conversely, pain at the injection site was the most common local symptom in the mRNA vaccine (RR 40.63, 95% CI: 5.85 to 282.44). Myalgia (RR 3.96, 95% CI: 2.35 to 6.66, I 2 = 29%), nausea (RR 3.74, 95% CI: 0.83 to 16.9, I 2 = 75%) and chill (RR 7.37, 95% CI: 4.20 to 12.94, I 2 = 0%) were the most common symptoms reported in recipients of adenovirus vaccine. Of note, the mRNA vaccine exhibited the highest risk of adverse effects graded as 3 or higher (RR 20.79, 95% CI: 1.30 to 333.14). No RSV prefusion F vaccine-related deaths were recorded in these studies.
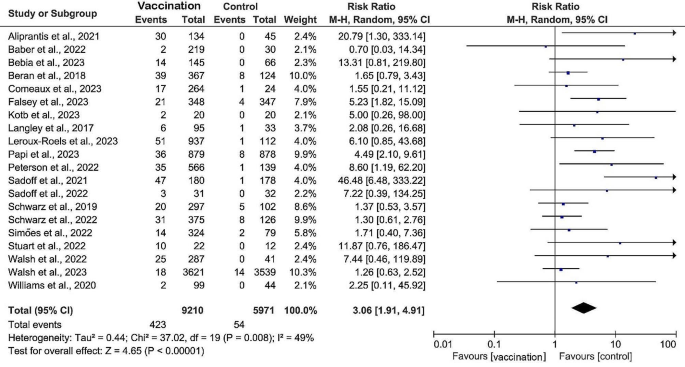
Incidence of grade ≥ 3 adverse events among the vaccination versus the control group
In this systematic review and meta-analysis of 22 studies, we explore for the first time the efficacy, immunogenicity, and safety of RSV prefusion F vaccine. We found that administration of the RSV prefusion F vaccine significantly reduced the risk of RSV-associated acute respiratory illness, particularly the risk of severe cases of RSV-associated lower respiratory tract illness requiring medical attention. Previous studies have found that vaccines using the fused RSV F protein as antigen, although immunogenic, do not prevent RSV-associated acute respiratory illness in the elderly, and there is no clinically identifiable patient population that may benefit from this vaccine [ 28 ]. The failure of these clinical studies has led to intensive investigation of the immune mechanism of RSV. Valuable experience has been accumulated for the development of safe and effective vaccines targeting the F prefusion protein of RSV. In eight studies involving the evaluation of vaccine efficacy, subunit vaccines appeared to provide better protection than adenovirus vaccines, but due to the limited number of studies of the two vaccines included in this study, further research remains imperative.
This study provides a comprehensive assessment of the available literature on RSV prefusion F vaccines. We found that existing subunit vaccines, adenovirus vaccines, mixed subunit and adenovirus vaccines, and mRNA vaccines were able to generate significant immune responses against RSV in vaccine recipients. The titers of neutralizing antibodies against RSV-A and RSV-B and RSV-specific ligation antibodies were significantly different among different vaccine types due to the differences in immunogenicity composition, whether they contained adjuvants or not, immunization dose, immunization times, and detection time. In our study, five studies used the ELISPOT assay to measure T-cell immune responses and showed that subunit vaccines elicited weaker T-cell responses than adenovirus vaccines, mixed subunit and adenovirus vaccines, and mRNA vaccines, which is consistent with the results of a large number of studies of COVID-19 vaccines [ 29 , 30 ].
Local adverse reactions after vaccination are more common than systemic adverse reactions. For different vaccine types, subunit vaccines are significantly safer and have lower incidence of local and systemic adverse reactions. Consistent with our results, the mRNA vaccine was associated with the highest incidence of adverse reactions except for a few [ 31 ]. In addition, mRNA vaccines have a higher association with serious adverse effects than other vaccine types [ 32 ]. Myalgia, nausea, and chills were the most common symptoms reported by adenovirus vaccine recipients, findings that were also similar to those previously reported for influenza and COVID-19 vaccines [ 30 ]. In theory, these differences could be attributed to differences in the strength of the immune response to the different vaccines [ 33 , 34 ], as confirmed by the efficacy and immunization results of this review.
In addition, there is concern about whether RSV vaccination can cause a potentially risky rare neurologic disorder (Guillain-Barre syndrome). While GBS is considered uncommon, it remains a significant subject of discussion in the context of vaccination. Previous research on influenza vaccination has reported an eightfold rise in the risk of GBS [ 35 ]. Similarly, investigations into COVID-19 vaccines have indicated diverse clinical associations between COVID-19 vaccination and GBS [ 36 , 37 ]. It is noteworthy that, reassuringly, there is currently no observed elevated risk of GBS associated with RSV vaccination.
This study has several limitations. First, current studies of RSV vaccine protection have been based on assessments of effectiveness during the first RSV season after vaccination (approximately 6 months). There were insufficient data to evaluate the duration of efficacy and immune effects after vaccination, and whether the vaccines result in long-term adverse events, thus necessitating long-term surveillance and study for the population. Second, the study included four vaccine types, but there was considerable variation in the number of studies across vaccine types. To eliminate this effect, we performed a subgroup analysis.
In conclusion, our meta-analysis suggests that vaccines using the RSV prefusion F protein as antigen exhibit favorable efficacy, immunogenicity, and safety in the population. In particular, it provides high protective efficiency against severe RSV-associated lower respiratory tract disease.
Data availability
The original contributions presented in the study are included in the article/supplementary material. Further inquiries can be directed to the corresponding author.
Langedijk AC, Bont LJ. Respiratory syncytial virus infection and novel interventions. Nat Rev Microbiol 2023.
Li Y, Wang X, Blau DM, Caballero MT, Feikin DR, Gill CJ, Madhi SA, Omer SB, Simões EAF, Campbell H, et al. Global, regional, and national disease burden estimates of acute lower respiratory infections due to respiratory syncytial virus in children younger than 5 years in 2019: a systematic analysis. Lancet. 2022;399(10340):2047–64.
Article PubMed PubMed Central Google Scholar
McLellan JS, Chen M, Joyce MG, Sastry M, Stewart-Jones GB, Yang Y, Zhang B, Chen L, Srivatsan S, Zheng A, et al. Structure-based design of a fusion glycoprotein vaccine for respiratory syncytial virus. Science. 2013;342(6158):592–8.
Article CAS PubMed PubMed Central Google Scholar
Krarup A, Truan D, Furmanova-Hollenstein P, Bogaert L, Bouchier P, Bisschop IJM, Widjojoatmodjo MN, Zahn R, Schuitemaker H, McLellan JS, et al. A highly stable prefusion RSV F vaccine derived from structural analysis of the fusion mechanism. Nat Commun. 2015;6:8143.
Article PubMed Google Scholar
Moher D, Shamseer L, Clarke M, Ghersi D, Liberati A, Petticrew M, Shekelle P, Stewart LA. Preferred reporting items for systematic review and meta-analysis protocols (PRISMA-P) 2015 statement. Syst Rev. 2015;4(1):1.
Aliprantis AO, Shaw CA, Griffin P, Farinola N, Railkar RA, Cao X, Liu W, Sachs JR, Swenson CJ, Lee H, et al. A phase 1, randomized, placebo-controlled study to evaluate the safety and immunogenicity of an mRNA-based RSV prefusion F protein vaccine in healthy younger and older adults. Hum Vaccines Immunotherapeutics. 2021;17(5):1248–61.
Article CAS Google Scholar
Baber J, Arya M, Moodley Y, Jaques A, Jiang Q, Swanson KA, Cooper D, Maddur MS, Loschko J, Gurtman A, et al. A phase 1/2 study of a respiratory Syncytial Virus Prefusion F Vaccine with and without adjuvant in healthy older adults. J Infect Dis. 2022;226(12):2054–63.
Bebia Z, Reyes O, Jeanfreau R, Kantele A, De Leon RG, Sánchez MG, Banooni P, Gardener GJ, Rasero JLB, Pardilla MBE, et al. Safety and Immunogenicity of an investigational respiratory Syncytial Virus Vaccine (RSVPreF3) in mothers and their infants: a phase 2 Randomized Trial. J Infect Dis. 2023;228(3):299–310.
Beran J, Lickliter JD, Schwarz TF, Johnson C, Chu L, Domachowske JB, Van Damme P, Withanage K, Fissette LA, David MP, et al. Safety and Immunogenicity of 3 formulations of an investigational respiratory Syncytial Virus Vaccine in Nonpregnant women: results from 2 phase 2 trials. J Infect Dis. 2018;217(10):1616–25.
Comeaux CA, Bart S, Bastian AR, Klyashtornyy V, De Paepe E, Omoruyi E, van der Fits L, van Heesbeen R, Heijnen E, Callendret B et al. Safety, Immunogenicity, and Regimen Selection of Ad26.RSV.preF-based vaccine combinations: a Randomized, Double-blind, Placebo-Controlled, phase 1/2a study. J Infect Dis 2023.
Falsey AR, Williams K, Gymnopoulou E, Bart S, Ervin J, Bastian AR, Menten J, De Paepe E, Vandenberghe S, Chan EKH, et al. Efficacy and safety of an Ad26.RSV.preF-RSV preF protein vaccine in older adults. N Engl J Med. 2023;388(7):609–20.
Article CAS PubMed Google Scholar
Kampmann B, Madhi SA, Munjal I, Simões EAF, Pahud BA, Llapur C, Baker J, Pérez Marc G, Radley D, Shittu E, et al. Bivalent Prefusion F vaccine in pregnancy to prevent RSV illness in infants. N Engl J Med. 2023;388(16):1451–64.
Kotb S, Haranaka M, Folschweiller N, Nakanwagi P, Verheust C, De Schrevel N, David MP, Mesaros N, Hulstrøm V. Safety and immunogenicity of a respiratory syncytial virus prefusion F protein (RSVPreF3) candidate vaccine in older Japanese adults: a phase I, randomized, observer-blind clinical trial. Respiratory Invest. 2023;61(2):261–9.
Langley JM, Aggarwal N, Toma A, Halperin SA, McNeil SA, Fissette L, Dewé W, Leyssen M, Toussaint JF, Dieussaert I. A Randomized, Controlled, Observer-Blinded phase 1 study of the Safety and Immunogenicity of a respiratory Syncytial Virus Vaccine with or without Alum Adjuvant. J Infect Dis. 2017;215(1):24–33.
Leroux-Roels I, Davis MG, Steenackers K, Essink B, Vandermeulen C, Fogarty C, Andrews CP, Kerwin E, David MP, Fissette L, et al. Safety and Immunogenicity of a respiratory Syncytial Virus Prefusion F (RSVPreF3) candidate vaccine in older adults: phase 1/2 Randomized Clinical Trial. J Infect Dis. 2023;227(6):761–72.
Papi A, Ison MG, Langley JM, Lee DG, Leroux-Roels I, Martinon-Torres F, Schwarz TF, van Zyl-Smit RN, Campora L, Dezutter N, et al. Respiratory Syncytial Virus Prefusion F protein vaccine in older adults. N Engl J Med. 2023;388(7):595–608.
Peterson JT, Zareba AM, Fitz-Patrick D, Essink BJ, Scott DA, Swanson KA, Chelani D, Radley D, Cooper D, Jansen KU, et al. Safety and Immunogenicity of a respiratory Syncytial Virus Prefusion F Vaccine when Coadministered with a Tetanus, Diphtheria, and Acellular Pertussis Vaccine. J Infect Dis. 2022;225(12):2077–86.
Sadoff J, De Paepe E, DeVincenzo J, Gymnopoulou E, Menten J, Murray B, Rosemary Bastian A, Vandebosch A, Haazen W, Noulin N, et al. Prevention of respiratory syncytial virus infection in healthy adults by a single immunization of Ad26.RSV.preF in a human challenge study. J Infect Dis. 2022;226(3):396–406.
Sadoff J, De Paepe E, Haazen W, Omoruyi E, Bastian AR, Comeaux C, Heijnen E, Strout C, Schuitemaker H, Callendret B. Safety and Immunogenicity of the Ad26.RSV.preF investigational vaccine Coadministered with an Influenza Vaccine in older adults. J Infect Dis. 2021;223(4):699–708.
Schmoele-Thoma B, Zareba AM, Jiang Q, Maddur MS, Danaf R, Mann A, Eze K, Fok-Seang J, Kabir G, Catchpole A, et al. Vaccine efficacy in adults in a respiratory Syncytial Virus Challenge Study. N Engl J Med. 2022;386(25):2377–86.
Schwarz TF, Johnson C, Grigat C, Apter D, Csonka P, Lindblad N, Nguyen TL, Gao FF, Qian H, Tullio AN, et al. Three dose levels of a maternal respiratory syncytial virus vaccine candidate are well tolerated and immunogenic in a Randomized Trial in Nonpregnant Women. J Infect Dis. 2022;225(12):2067–76.
Schwarz TF, McPhee RA, Launay O, Leroux-Roels G, Talli J, Picciolato M, Gao F, Cai R, Nguyen TL, Dieussaert I, et al. Immunogenicity and safety of 3 formulations of a respiratory syncytial virus candidate vaccine in Nonpregnant women: a phase 2, Randomized Trial. J Infect Dis. 2019;220(11):1816–25.
Simões EAF, Center KJ, Tita ATN, Swanson KA, Radley D, Houghton J, McGrory SB, Gomme E, Anderson M, Roberts JP, et al. Prefusion F protein-based respiratory Syncytial Virus immunization in pregnancy. N Engl J Med. 2022;386(17):1615–26.
Stuart A, Virta M, Williams K, Seppa I, Hartvickson R, Greenland M, Omoruyi E, Bastian AR, Haazen W, Salisch N et al. Phase 1/2a Safety and Immunogenicity of an Adenovirus 26 Vector RSV Vaccine Encoding Prefusion F in Adults 18–50 Years and RSV Seropositive Children 12–24 Months. Journal of infectious diseases 2022.
Walsh EE, Falsey AR, Scott DA, Gurtman A, Zareba AM, Jansen KU, Gruber WC, Dormitzer PR, Swanson KA, Radley D, et al. A Randomized Phase 1/2 study of a respiratory Syncytial Virus Prefusion F Vaccine. J Infect Dis. 2022;225(8):1357–66.
Walsh EE, Pérez Marc G, Zareba AM, Falsey AR, Jiang Q, Patton M, Polack FP, Llapur C, Doreski PA, Ilangovan K, et al. Efficacy and safety of a bivalent RSV prefusion F vaccine in older adults. N Engl J Med. 2023;388(16):1465–77.
Williams K, Bastian AR, Feldman RA, Omoruyi E, de Paepe E, Hendriks J, van Zeeburg H, Godeaux O, Langedijk JPM, Schuitemaker H, et al. Phase 1 safety and immunogenicity study of a respiratory Syncytial Virus Vaccine with an Adenovirus 26 Vector Encoding Prefusion F (Ad26.RSV.preF) in adults aged ≥ 60 years. J Infect Dis. 2020;222(6):979–88.
Falloon J, Yu J, Esser MT, Villafana T, Yu L, Dubovsky F, Takas T, Levin MJ, Falsey AR. An Adjuvanted, Postfusion F protein-based vaccine did not prevent respiratory Syncytial Virus illness in older adults. J Infect Dis. 2017;216(11):1362–70.
Al-Sheboul SA, Brown B, Shboul Y, Fricke I, Imarogbe C, Alzoubi KH. An immunological review of SARS-CoV-2 infection and vaccine serology: innate and adaptive responses to mRNA, Adenovirus, inactivated and protein subunit vaccines. Vaccines 2022;11(1).
McDonald I, Murray SM, Reynolds CJ, Altmann DM, Boyton RJ. Comparative systematic review and meta-analysis of reactogenicity, immunogenicity and efficacy of vaccines against SARS-CoV-2. NPJ Vaccines. 2021;6(1):74.
Ling Y, Zhong J, Luo J. Safety and effectiveness of SARS-CoV-2 vaccines: a systematic review and meta-analysis. J Med Virol. 2021;93(12):6486–95.
Al Khames Aga QA, Alkhaffaf WH, Hatem TH, Nassir KF, Batineh Y, Dahham AT, Shaban D, Al Khames Aga LA, Agha MYR, Traqchi M. Safety of COVID-19 vaccines. J Med Virol. 2021;93(12):6588–94.
Fang E, Liu X, Li M, Zhang Z, Song L, Zhu B, Wu X, Liu J, Zhao D, Li Y. Advances in COVID-19 mRNA vaccine development. Signal Transduct Target Ther. 2022;7(1):94.
Pollard AJ, Bijker EM. A guide to vaccinology: from basic principles to new developments. Nat Rev Immunol. 2021;21(2):83–100.
Schonberger LB, Bregman DJ, Sullivan-Bolyai JZ, Keenlyside RA, Ziegler DW, Retailliau HF, Eddins DL, Bryan JA. Guillain-Barre syndrome following vaccination in the National Influenza Immunization Program, United States, 1976–1977. Am J Epidemiol. 1979;110(2):105–23.
Chowdhury S, Chowdhury S. Association of Guillain-Barré syndrome following COVID-19 vaccination. Int J ImmunoPathol Pharmacol. 2023;37:3946320231199349.
Abara WE, Gee J, Marquez P, Woo J, Myers TR, DeSantis A, Baumblatt JAG, Woo EJ, Thompson D, Nair N, et al. Reports of Guillain-Barré Syndrome after COVID-19 vaccination in the United States. JAMA Netw Open. 2023;6(2):e2253845.
Download references
Acknowledgements
We also thanks for the support of Construction of Key Clinical Specialties in Guangxi (Guiweiyifa [2022]-NO.21) and the Key Laboratory of Molecular Pathology of Guangxi.
Not applicable.
Author information
Yi Pang and Haishan Lu contributed equally to this work.
Authors and Affiliations
Youjiang Medical University for Nationalities, Baise, China
Clinicopathological Diagnosis & Research Center, The Affiliated Hospital of Youjiang Medical University for Nationalities, Baise, China
Haishan Lu, Demin Cao, Xiaoying Zhu, Qinqin Long, Fengqin Tian, Xidai Long & Yulei Li
Key Laboratory of Tumor Molecular Pathology of Guangxi Higher Education Institutes, Baise, China
You can also search for this author in PubMed Google Scholar
Contributions
Y.P. and H.L. analyzed the data, and drafted and revised the manuscript. X.Z., D.C., Q.L. and F.T. retrieved and collected the data. X.L. and Y.L. supervised the study and approved the final manuscript. All authors have agreed to the published version of the manuscript. All authors read and approved the final manuscript.
Corresponding authors
Correspondence to Xidai Long or Yulei Li .
Ethics declarations
Ethical approval, consent for publication, competing interests.
The authors declare no competing interests.
Additional information
Publisher’s note.
Springer Nature remains neutral with regard to jurisdictional claims in published maps and institutional affiliations.
Electronic supplementary material
Below is the link to the electronic supplementary material.
Supplementary Material 1
Rights and permissions.
Open Access This article is licensed under a Creative Commons Attribution 4.0 International License, which permits use, sharing, adaptation, distribution and reproduction in any medium or format, as long as you give appropriate credit to the original author(s) and the source, provide a link to the Creative Commons licence, and indicate if changes were made. The images or other third party material in this article are included in the article’s Creative Commons licence, unless indicated otherwise in a credit line to the material. If material is not included in the article’s Creative Commons licence and your intended use is not permitted by statutory regulation or exceeds the permitted use, you will need to obtain permission directly from the copyright holder. To view a copy of this licence, visit http://creativecommons.org/licenses/by/4.0/ . The Creative Commons Public Domain Dedication waiver ( http://creativecommons.org/publicdomain/zero/1.0/ ) applies to the data made available in this article, unless otherwise stated in a credit line to the data.
Reprints and permissions
About this article
Cite this article.
Pang, Y., Lu, H., Cao, D. et al. Efficacy, immunogenicity and safety of respiratory syncytial virus prefusion F vaccine: systematic review and meta-analysis. BMC Public Health 24 , 1244 (2024). https://doi.org/10.1186/s12889-024-18748-8
Download citation
Received : 02 December 2023
Accepted : 30 April 2024
Published : 06 May 2024
DOI : https://doi.org/10.1186/s12889-024-18748-8
Share this article
Anyone you share the following link with will be able to read this content:
Sorry, a shareable link is not currently available for this article.
Provided by the Springer Nature SharedIt content-sharing initiative
- Respiratory syncytial virus
- Prefusion F vaccine
- Immunogenicity
BMC Public Health
ISSN: 1471-2458
- Submission enquiries: [email protected]
- General enquiries: [email protected]

IMAGES
VIDEO
COMMENTS
Two doses of the vaccine or placebo were given 21 days apart to the respective groups. 21 The mean age of the participants was 45.3 years, and the majority of participants were Caucasian (98.5%). 21 From 21 days after the first dose of the vaccine, efficacy against symptomatic COVID-19 illness was 91.6% (95% CI, 85.6-95.2%) with 16 confirmed ...
To date, coronavirus disease 2019 (COVID-19) becomes increasingly fierce due to the emergence of variants. Rapid herd immunity through vaccination is needed to block the mutation and prevent the emergence of variants that can completely escape the immune surveillance. We aimed to systematically evaluate the effectiveness and safety of COVID-19 vaccines in the real world and to establish a ...
Overall, the PHE study showed vaccine effectiveness after two doses of the BNT162b2 vaccine of 94% against the alpha variant and 88% against the delta variant. The corresponding percentages with ...
The protective effects of vaccination and prior infection against severe Covid-19 are reviewed, with proposed directions for future research, including mucosal immunity and intermittent vaccine boo...
Metrics. Vaccines have revolutionized modern medicine by preventing infectious diseases and safeguarding public health. This Collection showcases cutting-edge research on advancements in vaccine ...
Here, we report safety and efficacy findings from the phase 2/3 part of a global phase 1/2/3 trial evaluating the safety, immunogenicity, and efficacy of 30 μg of BNT162b2 in preventing Covid-19 ...
Vaccine development for emerging infectious diseases. Jean-Louis Excler, Melanie Saville, Seth Berkley &. Jerome H. Kim. Nature Medicine 27 , 591-600 ( 2021) Cite this article. 71k Accesses. 197 ...
Abstract. Since the outbreak of the COVID-19 pandemic, there has been a rapid expansion in vaccine research focusing on exploiting the novel discoveries on the pathophysiology, genomics, and molecular biology of the severe acute respiratory syndrome coronavirus 2 (SARS-CoV-2) infection. Although the current preventive measures are primarily ...
There is no question that the current vaccines are effective and safe. The risk of severe reaction to a COVID-19 jab, say researchers, is outweighed by the protection it offers against the deadly ...
2020 has been a difficult year for all, but has seen 58 vaccines against severe acute respiratory syndrome coronavirus 2 (SARS-CoV-2) be developed and in clinical trials,1 with some vaccines reportedly having more than 90% efficacy against COVID-19 in clinical trials. This remarkable achievement is much-needed good news as COVID-19 cases are currently at their highest daily levels globally.2 ...
Background Despite reliable evidence-based research supporting the COVID-19 vaccines, population-wide confidence and trust remain limited. We sought to expand prior knowledge about COVID-19 vaccine perceptions, while determining which population groups are at greatest risk for not getting a vaccine. Methods Study participants in the U.S. (79% female, median age group 46-60 years) were ...
Our analyses indicate that vaccine effectiveness generally decreases over time against SARS-CoV-2 infections, hospitalisations, and mortality. The baseline vaccine effectiveness levels for the omicron variant were notably lower than for other variants. Therefore, other preventive measures (eg, face-mask wearing and physical distancing) might be necessary to manage the pandemic in the long term.
The COVID-19 pandemic has created a new reality where individuals are faced with a previously unknown disease and its effects, providing a unique opportunity to investigate vaccine attitudes during a period of heightened disease salience. The present research reports findings from a longitudinal study conducted during the COVID-19 health crisis ...
COVID-19 vaccine hesitancy: the five Cs to tackle behavioural and sociodemographic factors. J R Soc Med 2021;114:295-298. Waning vaccine efficacy remains greatest in older individuals and higher-risk groups. Lin DY, Gu Y, Wheeler B, Young H, Holloway S, Sunny SK, et al. Effectiveness of COVID-19 vaccines over a 9-month period in North Carolina.
The Coronavirus Efficacy (COVE) phase 3 trial was launched in late July 2020 to assess the safety and efficacy of the mRNA-1273 vaccine in preventing SARS-CoV-2 infection. An independent data and ...
By examining COVID-19 and influenza vaccinations as indicators, our research unveils a positive correlation between community notification efforts and both types of individual immunizations ...
17:15 - 17:25. WHO Target Product Profile for COVID Vaccines: current considerations. Ana María Henao-Restrepo. 17:25 - 17:35. Overview of various global efforts towards the identification of a protective level. Peter Dull. 17:35 - 17:45. Immunobridging to evaluate vaccines (different doses and target populations) Doran Fink.
WHO and its partners are committed to accelerating the development of COVID-19 vaccines while maintaining the highest standards on safety. Vaccines go through various phases of development and testing - there are usually three phases to clinical trials, with the last one designed to assess the ability of the product to protect against disease ...
A good research paper starts with a great topic, and we are here to help you nail that. We understand the significance of research papers, and that is why we have handpicked 170 out-of-the-box vaccination research paper topics, titles, and ideas to make your work seamless. Debate Topics About Vaccination. What is reverse vaccinology?
Tetanus vaccines: WHO position paper, February 2017 — recommendations. Vaccine 36, 3573-3575 ... (SAGE) and a National Institute for Health Research (NIHR) Senior Investigator. The views ...
A notable research gap exists in the systematic review and meta-analysis concerning the efficacy, immunogenicity, and safety of the respiratory syncytial virus (RSV) prefusion F vaccine. We conducted a comprehensive search across PubMed, Embase, the Cochrane Central Register of Controlled Trials, and ClinicalTrials.gov to retrieve articles related to the efficacy, immunogenicity, and safety of ...
This paper tackles the complex interplay between Human Immunodeficiency virus (HIV-1) and Mycobacterium tuberculosis (M. tuberculosis) infections, particularly their contribution to immunosenescence, the age-related decline in immune function. Using the current literature, we discuss the immunological mechanisms behind TB and HIV-induced immunosenescence and critically evaluate the BCG ...